Abstract
A theoretical model describing the dynamic process of a continuous-wave Fe2+:ZnSe laser is presented. The influence of some of the operating parameters on the output characteristics of an Fe2+:ZnSe laser is studied in detail. The results indicate that the temperature rise of the Fe2+:ZnSe crystal is significant with the use of a high power pump laser, especially for a high doped concentration of crystal. The optimal crystal length increases with decreasing the doped concentration of crystal, so an Fe2+:ZnSe crystal with simultaneous doping during growth is an attractive choice, which usually has a low doped concentration and long length. The laser pumping threshold is almost stable at low temperatures, but increases exponentially with a working temperature in the range of 180 K to room temperature. The main reason for this phenomenon is the short upper level lifetime and serious thermal temperature rise when the working temperature is higher than 180 K. The calculated optimum output mirror transmittance is about 35% and the performance of a continuous-wave Fe2+:ZnSe laser is more efficient at a lower operating temperature.
Export citation and abstract BibTeX RIS
1. Introduction
Mid-infrared lasers have attracted much attention for wide applications such as eye-safe laser radar, trace gas analysis, environmental monitoring, spectroscopy, laser communication, and numerous military applications. The transition metal iron doped ZnSe (Fe2+:ZnSe) laser has a broad absorption spectrum (helpful for broadband pumping) and broad fluorescence spectrum (helpful for broadband tuning), which is an innovative solid state laser in the mid-infrared range of 4–4.5 µm [1–3].
In recent years, solid state Fe2+:ZnSe lasers excited by a high power flash-lamp-pumped Er:YAG laser and a high-energy non-chain pulsed HF/DF laser have developed rapidly [4–10]. In 2015, using a 15 J flash-lamp-pumped Er:YAG laser as the excitation source, Velikanov demonstrated an Fe2+:ZnSe laser with a 4.9 J single pulse energy at 85 K, with a laser slope efficiency of 51% [4]. Later, Velikanov replaced the excitation source with a non-chain pulsed HF laser and obtained an Fe2+:ZnSe laser with a pulse energy of 1.2 J at room temperature [5]. Also in 2015, Martyshkin reported a repetition rate Fe2+:ZnSe laser with a maximum average power of 35 W at 77 K [6]. Both the flash-lamp-pumped Er:YAG laser and the non-chain pulsed HF/DF laser pump source have the disadvantages of large volume and low wall-plug efficiency [11, 12]. However, solid state continuous-wave Fe2+:ZnSe lasers using an LD pumped Er:YAG laser as the excitation source have the advantages of compact size and high wall-plug efficiency, which would have prospects in the future.
Sennaroglu reported a theoretical model of a continuous-wave Cr2+:ZnSe laser, and the output power performance was accurately simulated by the model [13]. There are some similarities between the energy level structure and the radiation mechanism of Fe2+:ZnSe and Cr2+:ZnSe lasers. The influence of the temperature rise on the fluorescence lifetime of a Cr2+:ZnSe laser is small with a low power pump, so the temperature factor is neglected in Sennaroglu's model [13]. However, the case of the Fe2+:ZnSe laser is different, as the temperature rise has a significant impact on fluorescence lifetime [3]. In this paper, taking into account the influence of the temperature rise caused by the laser pump on the fluorescence lifetime of an Fe2+:ZnSe laser, the theoretical model that can describe the laser dynamic process of a continuous-wave Fe2+:ZnSe laser is presented. The influence of temperature rise of crystal, crystal length, doped concentration of crystal, and output mirror transmittance on the performance of an Fe2+:ZnSe laser is studied by this model. The simulated output power of a continuous-wave Fe2+:ZnSe laser is consistent with the experimental result reported by Evans [14].
2. Model of continuous-wave Fe2+:ZnSe laser
The modified four-level energy diagram used in the model of the continuous-wave Fe2+:ZnSe laser is shown in figure 1. Pump photons at wavelength λp are absorbed by Fe2+ ions which then are excited from the ground state to the first excited state
. The energy level lifetime of
is much shorter than the upper level
. Decay from
to
is assumed to occur very rapidly, leaving approximately no ions on level
in the steady state. Ions on level
can either come down to
by stimulated and spontaneous emission or get promoted to the higher energy level
as result of excited-state absorption. Finally, the rapid decay from
to
can be assumed as leaving level
empty.
Figure 1. Energy level diagram of an Fe2+:ZnSe gain medium.
Download figure:
Standard image High-resolution imageThe absorption and the emission bands of the Fe2+:ZnSe laser overlap in the 3.5–5 µm spectral range at room temperature [15], so some laser photons at wavelength λl are absorbed by ground-state Fe2+ ions which are the excited to the upper level . For the short fluorescence lifetime of Fe2+ ions at room temperature, the Fe2+ ions on the upper level come down to the lower level and radiate laser photons at wavelength λl simultaneously. During this process, the absorbed and radiated photons have the same wavelength, so the quantum efficiency is about 1. Therefore, the process of absorption from the ground state at laser wavelength λl is neglected in this model at room temperature. The temporal evolution of the ion density N2 on upper level
can be described by the following rate equation:
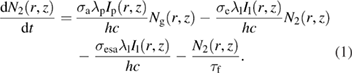
Here, Ng(r, z) is the ion density of ground state ; Ip(r, z) is the pump intensity; Il(r, z) (Il(r, z) =
(r, z) +
(r, z)) is the total cavity laser intensity at λl; h is the Planck constant; σa is the absorption cross-section at λp; σe is the emission cross-section; σesa is the excited-state absorption cross-section at λl; λp and λl are the pump and laser wavelengths, respectively, and τf is the fluorescence lifetime, which is a function of temperature.
We assume that the decay from level to
occurs very rapidly so that N4 is negligible in the steady state. Hence, to a very good approximation, dN2/dt = −dNg/dt and Nt = Ng (r, z) + N2(r, z). Under these approximations, it can be shown that in the steady state, the expression of N2 becomes:

where f (f = σesa/σe) is the normalized excited-state absorption strength at the laser wavelength λl. Isa (Isa = hc/σaλpτf) and Ise (Ise = hc/σaλlτf) are the absorption and emission saturation intensities, respectively.
In the steady state, stimulated absorption from the ground state leads to the attenuation of pump intensity, while stimulated emission from the upper laser level amplifies the circulating laser intensity. The propagation of Ip, Il in the resonator consisting of M1, M2 is shown in figure 2.
Figure 2. Schematic layout of laser propagation in the resonator.
Download figure:
Standard image High-resolution imageIn the crystal of Fe2+:ZnSe, under the condition of ignoring the atmospheric absorption of the pump laser (Ip1 = Ip0), the axial pump laser intensity Ip can be given by the differential equation:

where ap0 (ap0 = σa Ng) is the small-signal differential absorption coefficient at the pump wavelength λp. Meanwhile, ignoring the atmospheric absorption and scattering of the laser, we assume that =
,
=
,
=
= R1
,
=
,
= R2
. Above R1, R2 is the reflectance of the cavity mirrors. In the crystal of Fe2+:ZnSe, the axial laser intensity Il can be given by the differential equation:

where gt (gt = σeNt) is the maximum extractable small-signal differential gain coefficient and al0 is the small-signal differential absorption coefficient at the laser wavelength λl. By solving the above simultaneous equations, the laser intensity in the cavity can be obtained. Then, the output power can be calculated by Pout = A(1 − R2), where A is the laser cross-sectional area in the cavity.
Fedorov performed a least-squares fit of the lifetime data for an Fe2+:ZnSe crystal using the standard Mott model [16]:

where τrad and τNR are the radiative and non-radiative lifetimes, respectively; ΔEa is the energy gap between the intersection of the adiabatic potentials of the ground and excited states and the minimum of adiabatic potential of the excited state; Wa is the relaxation parameter, and kb is the Boltzmann's constant.
The effect of temperature on the cross-sections of absorption and emission are introduced in the model. The absorption cross-section spectra are obtained from absorption measurements [1], and different absorption cross-sections are used in the calculation. Emission cross-sections can be determined using the Fuchtbauer–Landenburg (FL) equation. Using the fundamental relationship between the spontaneous and stimulated processes, the FL equation can be written as:

where n is the refractive index, and c is the speed of light.
The temperature of the crystal has a significant influence on the fluorescence lifetime, so the temperature rise of the crystal caused by the laser pump affects the output power of the Fe2+:ZnSe laser. By neglecting longitudinal heat conduction and by approximating the heat load as a square function, the temperature distribution T1 (z) inside the crystal can be given by the following expression [13]:

Here, Tb is the boundary temperature; r0 is the effective crystal radius; and κ is the heat conductivity. The radial width wh(z) and the axial value h0(z) of the square heat load are further given by the following expressions:


where ωp(z) is the pump beam diameter and ηh is the heating coefficient. ηh gives the fraction of the absorbed pump energy that is converted to heat inside the crystal. Since the quantum efficiency of the Fe2+:ZnSe is near unity at a lower temperature, ηh is approximated as (1 − λp/λl).
3. Simulation results and discussion
3.1. Temperature rise of the crystal
The working temperature of laser crystal influences the lifetime of the upper laser energy. Especially at room temperature, the lifetime of the upper laser energy will rapidly decrease with the rise in temperature [16]. The serious temperature rise of the crystal caused by the laser pump leads to a temperature quenching effect in the Fe2+:ZnSe laser. Before calculating the relationship between the output performance of the continuous wave Fe2+:ZnSe laser and the temperature of the crystal, the temperature rise caused by the laser pump is calculated using equation (7). The calculated variables are listed in table 1.
Table 1. Values of the parameters of the Fe2+:ZnSe laser.
Definition | Parameter | Value/units |
---|---|---|
Energy gap | ΔEa | 1900 cm−1 [16] |
Relaxation parameter | 1/Wa | 5 ns [16] |
Normalized excited-state absorption strength | σesa/σe | 0.17 [17] |
Absorption cross-section | σa | 1.3 × 10−18 cm2 at lower temperature [1] |
0.97 × 10−18 cm2 at room temperature [1] | ||
Wavelength of pump beam | λp | 2.94 µm |
Pump beam waist | ω | 0.3 mm |
Wavelength of laser beam | λl | 4.1 µm |
Heat conductivity | κ | 0.19 W cm−1 K−1 [17] |
Absorption coefficient | al0 | 0.1 cm−1 |
Doped concentration | Nt | 5 × 1018 cm−2 |
Refractive index | n | 2.4 |
Crystal radius | R0 | 3 mm |
The absorption intensity of the pump laser is a function of the doped concentration of the Fe2+:ZnSe crystal. The relationship between the temperature rise at the incidence face of the crystal and the pump power is calculated at a doped concentration of 1 × 1018 cm−2, 5 × 1018 cm−2, and 10 × 1018 cm−2, respectively, and the results are shown in figure 3. The temperature at the incidence face increases linearly with the rise in pump power, and the higher the doped concentration, the higher the temperature rise of the crystal. The axial temperature rise at the maximum incident pump power of 10 W is approximately 20 K and 8 K with doped concentrations of 10 × 1018 cm−2 and 1 × 1018 cm−2, respectively. The experimental results of the lifetime temperature in [16] shows that the fluorescence lifetime deviates by less than 5% with the temperature rise of 20 K when the working temperature changes from 77–180 K. Since this deviation is very small, the influence of temperature on fluorescence lifetime is neglected in modeling the power performance of the continuous-wave in the temperature range of 77–180 K. When the working temperature is higher than 180 K, the fluorescence lifetime deviates by approximately 100% with a temperature rise of 20 K, so the influence of temperature on fluorescence lifetime cannot be neglected under this condition.
Figure 3. Calculated variation of the average axial temperature rise T1(z0)-Tb as a function of the incident pump power.
Download figure:
Standard image High-resolution image3.2. Laser threshold
After injecting the pump laser into the Fe2+:ZnSe crystal, and benefiting from the four-level energy structure, the population conversion between the upper and lower energy levels can be easily obtained. The laser grows due to the effect of the laser gain (stimulated amplification). Meanwhile, the laser weakens due to the effect of the loss (absorption loss and output coupling loss). Finally, whether the laser can be propagated or not depends on the values of gain and loss. Using the parameters listed in table 1, by solving equations (3) and (4), the relationship between laser intensity and crystal length in the cavity is calculated with different doping concentrations, and the results are shown in figure 4. Firstly, the laser intensity rapidly rises with the increment of crystal length and reaches the maximum. Then, for the weakening of the pump intensity and absorption loss at the longer crystal end, the laser intensity reduces gradually. The results show that the optical crystal length is about 13.6 mm, 7.9 mm, and 5.1 mm with a doped concentration of 1 × 1018 cm−2, 5 × 1018 cm−2, and 10 × 1018 cm−2, respectively. Taking into account the fact that the crystal with low doped concentration has a high temperature rise, an Fe2+:ZnSe single-crystal with simultaneous doping during growth is an attractive choice, which usually has a low doped concentration and long length.
Figure 4. Laser intensity as a function of crystal length at different doped concentrations.
Download figure:
Standard image High-resolution imageTaking into account the influence of the temperature rise caused by the pump laser on the level lifetime of the Fe2+:ZnSe laser, the relationship between the laser threshold and the temperature of the crystal is calculated at different doping concentrations. During the calculation, the crystal length is set at an optimal value at each doping concentration. The calculation is shown in figure 5. When the temperature is lower than 180 K, the laser threshold (about 0.2 W) is invariable at each doping concentration, which is in agreement with the experimental results reported by Evans [14]. When the temperature is higher than 180 K, the temperature has an obvious influence on the threshold of the Fe2+:ZnSe laser and the laser threshold increases exponentially with the rise in the working temperature. Under the condition of the same pump power, the higher the doped concentration, the higher the laser threshold. At room temperature, the calculated laser threshold is about 20 W, 24 W, and 29 W with doped concentrations of 1 × 1018 cm−2, 5 × 1018 cm−2, and 10 × 1018 cm−2, respectively.
Figure 5. Laser threshold as a function of temperature at different doped concentrations.
Download figure:
Standard image High-resolution image3.3. Output characteristics of the continuous wave Fe2+:ZnSe laser
Under continuous wave pumping, the population of the upper level is invariable with the combined effect of stimulated absorption and emission, so the continuous wave Fe2+:ZnSe laser has a stable output power. By simultaneously solving equations (2)–(4), using the parameters listed in table 1, the relationship between the laser output power and the pump power is calculated, and the results are shown in figure 6. When the temperature is 77 K, ignoring the influence of the temperature rise on the level lifetime, the output power of the Fe2+:ZnSe laser increases linearly with the pump power, and the laser slope efficiency is about 42%. This simulation is in good agreement with the experiment reported by Evans [14], which shows that the model can be used to analyze the laser dynamic process of a continuous-wave Fe2+:ZnSe laser. By using thermoelectric coolers, a lower working temperature can easily reach 233 K, where the influence of the temperature rise on the level lifetime should not be ignored. The output power of the Fe2+:ZnSe laser increases with pump power, but the laser slope efficiency drops to 25%. And the laser efficiency further drops when the pump power is higher than 9 W. At room temperature, it is hard to operate a continuous-wave Fe2+:ZnSe laser. Now, scaling up the output power of the continuous-wave Fe2+:ZnSe laser is limited by the pump laser power at a lower temperature. While short level lifetime and serious thermal temperature rise are the key factors in limiting the operation of the Fe2+:ZnSe laser operation at room temperature.
Figure 6. Fe2+:ZnSe laser output power as a function of pump power at temperature of 77 K and 233 K.
Download figure:
Standard image High-resolution imageIn order to determine the optimal transmittance of the output mirror, the above procedure is repeated as a function of output mirror transmittance. At a temperature of 77 K and a pump power of 2 W, the relationships among the laser power, optical efficiency and transmittance of output mirror are calculated, and the results are shown in figure 7. The laser power, optical efficiency, and transmittance of the output mirror are in a quadratic curve. The optimal transmittance is about 35%, and the maximum laser power and optical efficiencies are 0.78 W and 39%, respectively.
Figure 7. Fe2+:ZnSe laser output power and optical efficiency as a function of transmittance.
Download figure:
Standard image High-resolution image4. Conclusion
A theoretical model has been built to study the characteristics of a continuous wave Fe2+:ZnSe laser, and the temperature rise of crystal caused by a pump laser is calculated. Under the temperature range of 77 K–180 K, with an optimal crystal length, the doped concentration and the temperature rise have little effect on the output performance of the Fe2+:ZnSe laser. The laser threshold is about 0.2 W, and the laser slope efficiency is about 42% at 77 K, which is the optimal working temperature for a continuous-wave Fe2+:ZnSe laser. When the temperature is higher than 180 K, the doped concentration and the temperature rise have an obvious impact on the output performance of the Fe2+:ZnSe laser. At 233 K, which can be easily reached by using thermoelectric coolers, the laser threshold and laser slope efficiency are about 4.5 W and 25%, respectively. At room temperature, it is hard to operate the continuous-wave Fe2+:ZnSe laser due to its high laser threshold and serious thermal temperature rise.
Acknowledgments
This work is supported by the International Cooperation Special Fund from Ministry of Science and Technology, PRC (2016YFE0120200), the National Natural Science Foundation of China (61705219), the Key Science and Technology Project Bidding of Jilin Province (20160203016GX), and the Science and Technology Innovation Talents Project of Jilin Province (20170519012JH).