Abstract
We have investigated the magnetocaloric effect in double perovskite Gd2NiMnO6 (GNMO) and Gd2CoMnO6 (GCMO) samples by magnetic and heat capacity measurements. Ferromagnetic ordering is observed at ~130 K (~ 112 K) in GNMO (GCMO), while the Gd exchange interactions seem to dominate for T < 20 K. In GCMO, below 50 K, antiferromagnetic behaviour due to the 3d–4f negative exchange interaction is observed. A maximum entropy (−ΔSM) and adiabatic temperature change of ~35.5 J Kg−1 K−1 (~ 24 J Kg−1 K−1) and 10.5 K (6.5 K) is observed in GNMO (GCMO) for a magnetic field change of 7 T at low temperatures. Absence of magnetic and thermal hysteresis and their insulating nature make them promising for low temperature magnetic refrigeration.
Export citation and abstract BibTeX RIS
1. Introduction
Magnetic refrigerant materials exhibit a temperature change when exposed to adiabatic demagnetization. The phenomena is known as solid-state magnetic refrigeration [1, 2] and strong coupling of the magnetic moment with lattice thermal energy is desirable in such materials. Magnetic refrigeration is an emerging technology alternative to the conventional gas-compression refrigeration in food preservation and air conditioning applications. Importantly, solid state cooling offers noise-free and energy efficient refrigeration suitable for room temperature cooling and cooling of microelectronic components [2–4]. Solid state cooling is essential for the growing needs of low temperature applications in space, particle detectors, and medical applications [2, 3, 5–7]. Liquefaction of H2 (20 K) by magnetocaloric method has been reported to be cost effective and could render hydrogen to be a competitive alternative fuel [8]. To achieve cooling below 1 K, the adiabatic demagnetization refrigeration is an attractive process compared to 3He/4He dilution refrigeration because of the growing cost of helium and scarcely available 3He isotope.
For low temperature magnetic refrigerant materials, it is important to have: (i) large effective spin quantum number, (ii) low magnetic anisotropy and low magnetic ordering temperature, (iii) small specific heat (iv) large magnetization under magnetic field, and (v) weak magnetic exchange interactions [9]. In magnetic refrigeration technology, Gd and Gd based alloys [10, 11], large molecular materials [12] have continued to receive large attention because large magnetic moment of Gd. Recently, R2BMnO6 (R = rare earth, B = Ni, Co) ceramics have attracted extensive research attention due to their ferromagnetic insulating behaviour with large magneto-dielectric effect and ferroelectric characteristics [13–15]. Coexistence of magnetocaloric and electrocaloric effects with multicaloric coupling in Y2CoMnO6 has excited the role of double perovskites in solid state refrigeration applications [16]. In this study we present the occurrence of large magnetic entropy change ~35.5 J Kg−1 K−1 and ~24 J Kg−1 K−1 in double perovskite GNMO and GCMO samples respectively; from heat capacity, a large adiabatic temperature change of ~10.5 K at low temperature has been noticed in GNMO. These values are much larger than the parent La2NiMnO6 and La2CoMnO6 compounds [17, 18].
2. Experimental details
Polycrystalline Gd2BMnO6 (B = Ni and Co) samples were prepared by solid-state reaction method using high purity precursor materials of Gd2O3, NiO, Co3O4 and MnO2. Stoichiometric proportion of the powders were thoroughly mixed and heated at temperatures from 1000 °C to 1350 °C with an intermediate grindings until the desired phase was obtained. The structural analysis was done using a Phillips powder x-ray diffractometer with Cu-Kα radiation. Rietveld refinement has revealed monoclinic crystal structure of P21/n space group for both the samples similar to other double perovskite compounds [13–15, 19]. Magnetic measurements were done with an EverCool Quantum Design SQUID-VSM magnetometer. The heat capacity at different constant magnetic fields was measured using relaxation method in a Quantum design-physical property measurement system (PPMS).
3. Results and discussion
Figures 1(a) and (b) shows the temperature dependent magnetization, M (T) in zero-field cooled (ZFC) and field-cooled (FC) modes with 0.01 T dc field for both the samples. In GNMO, a paramagnetic (PM) to ferromagnetic (FM) ordering is observed at TC ~ 132 K and a rise in MFC at low temperatures (T < 20 K) can be attributed to the polarization of Gd spins with Gd3+– O – Gd3+ exchange interactions. In case of GCMO, PM to FM phase transition is noticed at TC ~ 112 K and it is followed by a broad peak around ~47 K suggesting the polarization of magnetic moments with an antiferromagnetic (AFM) like behaviour. At low temperatures, an increasing magnetization due to Gd can be found similar to GNMO.The FM ordering can be attributed to the super exchange interaction of Ni2+− – O –Mn4+ (Co2+ −– O –Mn4+) magnetic species in GNMO (GCMO). The AFM arrangement of magnetic moments at ~47 K in GCMO can be ascribed to the negative 3d–4f exchange interactions in between FM network of Co/Mn sublattice and Gd spins, like in RMnO3 and RCrO3 (R = Gd, Ho, and Dy) systems [20–23]. We have observed no bifurcation in between field-cooled cooling and field-cooled warming modes of magnetization (not shown here) that suggests the absence of thermal hysteresis in both the samples.
Figure 1. M versus T (K) data in ZFC (solid symbol), FC (open symbol) modes for 0.01 T field for (a) GNMO and (b) GCMO; and their inset shows the CW law fit to the experimental data of χ−1 versus T (K), (c) and (d) shows the Cp versus T (K) data for different magnetic fields in GNMO and GCMO samples respectively, and their inset displays the magnified view of CP versus T (K) data at T < 40 K.
Download figure:
Standard image High-resolution imageTemperature dependent magnetic susceptibility (χ)in PM state is fitted to Curie–Weiss (CW) law with an additional susceptibility contribution of Gd magnetic moments and effective χ given as,

here, C(Ni/Co)–Mn and CGd are the Curie constants of (Ni/Co)–O–Mn network and Gd sublattices respectively. Inset to figures 1(a) and (b) shows the χ−1 versus T data of GNMO and GCMO samples and the fit to the equation (1) is shown by the solid line. The Curie constant is estimated using the formula,
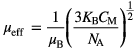
where, is the Boltzmann constant and μB is Bohr magneton. From the fitting, in GNMO sample, the obtained CW temperature (θ) = 145 K and the effective PM moments (μeff)Ni–Mn and (μeff)Gd are ~6.37 μB and ~13.2 μB respectively. In GCMO, θ ~ 111.2 K and (μeff)Co–Mn ~ 5.81 μB and (μeff)Gd ~ 14.49 μB. In both the samples, the effective PM moment values are close to the theoretically calculated spin only contribution of (Ni/Co)–O–Mn network and rare-earth Gd spins.
Thermal evolution of heat capacity (CP) of GNMO and GCMO samples under different magnetic fields (i.e. 0, 1, 3, 5 and 7 T) are depicted in figures 1(c) and (d) respectively. The CP versus T displays distinct transitions in both the samples. A λ-type anomaly under zero field at ~130 K in GNMO and ~112 K in GCMO samples correspond to the second-order magnetic phase transition. In both the samples, with the increase of magnetic field, magnitude of the peak decreases and shifts to high-temperature side which is the characteristic feature of FM ordering. The broadening of CP (T) peak under external field is due to the randomization of magnetic moments in wide temperature region. Above 20 K, CP (T) increases with increasing temperature and follows the T3 dependence due to the lattice contribution [24], and all CP versus T curves gets merged for different magnetic fields as shown in the inset of figures 1(c) and (d). The increasing trend in heat capacity below ~20 K can be noticed in both GNMO and GCMO samples and can be attributed to the Gd magnetic contribution. With the application of magnetic field, CP (T) value increases and broadens the dip at ~20 K and shifts to higher temperature; this anomalous behaviour can be attributed to the schottkey contribution that arises from the splitting of degenerate ground state energy levels at the Gd3+ state in crystal fields [25, 26]. In contrast to GNMO sample, a peak in CP (T) can be noticed at ~5 K in GCMO corresponding to the onset of AFM ordering of Gd magnetic moments. In GNMO, this peak can be noticed only with the magnetic fied and this suggests the Gd ordering occurs at temperatures lower than 2 K.
Isothermal field-dependent magnetization i.e. M (H) of GNMO and GCMO samples at 2 K is shown in the figure 2(a). Both the samples show no hysteresis and such kind of magnetic reversibility in M (H) is beneficial for the solid-state magnetic refrigeration. GNMO shows S-shaped M (H) behaviour with significant changes in magnetization at low fields and saturation like behaviour for H ⩾ 6 T. The observed saturation magnetization (MS) value of ~18.9 μB/f.u. matches well with the theoretically estimated value of 19 μB/f.u (5 μB/f.u for Ni2+–O–Mn4+ pair and 14 μB/f.u for Gd2). While GCMO exhibits a linear variation of magnetization from low fields to 4 T (see inset of figure 2(a)) and shows no saturation even for H ⩾ 6 T. Further, in GCMO, the magnetization value at high field (7 T in our set up) is ~16.5 μB/f.u., which is smaller than theoretically estimated sum of the fully polarized Co2+–O–Mn4+ interaction (6 μB/f.u.) and magnetic moment of Gd spins (14 μB/Gd2). Incomplete saturation of magnetization in GCMO can be related to the presence of significant 3d–4f negative AFM exchange correlations. Figures 2(b) and (c) shows the representative isothermal M (H) plots of GNMO and GCMO samples taken in the temperature range of 2–40 K. With this data, we have calculated MCE using Maxwell's relation [27],
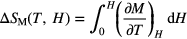
Figure 2. (a) Isothermal M (H) loops at 2 K for GCMO and GNMO samples, inset shows the blown up portion of the hysteresis loop at low field region, (b) and (c) are representative M (H) isotherms at selective temperatures for GNMO and GCMO samples respectively.
Download figure:
Standard image High-resolution imageSince, isothermal M (H) curves are measured by discrete field changes, the following expression is used,

here, Mi and Mi+1 are initial magnetization values at Ti and Ti+1 respectively for a field change of In this method, the magnetic entropy change corresponding to the average temperature T (= (T1 + T2)/2) is given by the area enclosed by two consecutive isothermal M (H) curves at T1 and T2 divided by ΔT = T2 − T1 (T2 > T1). We have calculated magnetic entropy change (−ΔSM) using equation (2) and is plotted with temperature for different magnetic fields as shown in the figures 3(a) and (b) for GNMO and GCMO samples respectively. The value of −ΔSM is positive in the entire temperature region and increases with the magnetic field; this indicates that the magnetic field favors FM ordering. In GNMO, −ΔSM increases with the decrease of temperature and a maximum change of entropy of ~35.5 J Kg−1 K−1 is observed at 2 K. In GCMO, a peak in −ΔSM can be noticed at ~5 K that corresponds to the AFM ordering of Gd and it is consistent with the heat capacity data in figure 1(d). The maximum change of entropy
(peak maximum at 4 K) is ~24 J Kg−1 K−1 for field change (ΔH) of 7 T. Theoretically, entropy is associated with the magnetic degrees of freedom and can be calculated using ΔSM = Rln (2S + 1), where R is the universal gas constant and S is the total spin quantum number and they are 40.68 J Kg−1 K−1 and 41.84 J Kg−1 K−1 for GNMO and GCMO respectively. Since the lattice contribution to the total entropy is negligible at low temperature, the maximum value of MCE calculated at 2 K is 82% in GNMO and 56% in GCMO with respect to the theoretically estimated magnetic entropy. Low value of
in GCMO is attributed to the incomplete saturation and low value of magnetization as noticed from M (H) (figure 2(a)). Moreover, the negative exchange coupling between (Co–O–Mn) and Gd sublattices can also be an effective cause for the low value of MCE. The MCE values of GNMO are larger than the paramagnetic salts [28, 29] and HoMn2O5 single crystal [30], while it is comparable to the Gadolinium Gallium Garnets Gd3(Ga1−xFex)5O12 [24], Gd and 3d-transition metal based small molecular magnetic systems [31], rare-earth manganites (RMnO3, R = Ho, Tb, Gd, Dy and Yb) [32, 33], and magnetically frustrated EuHo2O4, EuDy2O4 compounds [34]. Interestingly all these systems have similar magnetic ordering below the liquid hydrogen temperatures (~ 20 K). MCE has also been calculated from the heat capacity measurements using the following thermodynamic relation,


here, CP (H) and CP (0) are the heat capacity values measured with field and without field respectively. The entropy change from heat capacity data is shown in the inset of figures 3(a) and (b). Here, maximum values of −ΔSM and its behaviour calculated from complementary experimental tools have shown a close resemblance. From the heat capacity measurement, a small change in entropy has also been found near to FM-PM (TC) transition in both the samples; −ΔSM ~ 3 J Kg−1 K−1 and ~2 J Kg−1 K−1 in GNMO and GCMO respectively.
Figure 3. − ΔSM versus T (K) curves measured from the isothermal magnetization curves in (a) GNMO, (b) GCMO samples and their inset shows −ΔSM versus T (K) data measured from the heat capacity.
Download figure:
Standard image High-resolution imageApart from change in entropy, the total adiabatic temperature change, ΔTad associated with external magnetic field is an another important parameter for evaluating MCE materials and it is calculated from the temperature dependence of the total entropy [11]. The zero magnetic field corresponding to total entropy S (0, T) can be calculated by using heat capacity data as,


and magnetic field induced total entropy, S (H, T) is calculated by subtracting the corresponding −ΔSM from S (0, T ). Then, ΔTad value is estimated from the isoentropic difference in between the entropy curves S (0, T) and S (H, T ). Figure 4 shows the temperature dependent ΔTad under different magnetic fields for GNMO and GCMO (inset) samples. ΔTad shows a peak near FM transition with a magnitude of ~1.8 K in GNMO and ~0.8 K in GCMO samples for a field change of 7 T. At low temperatures <20 K with the onset of Gd ordering ΔTad shows a peak value of ~10.5 K in GNMO and ~6.5 K for GCMO samples. We have measured the electrical resistivity in these samples, and it is of the order of 106 Ωm at 150 K and it increases further with the decrease of temperature. Particularly, for low temperature refrigeration, high electric resistivity is desirable as the low resistivity of the materials can induce significant eddy current loss that limits the cooling efficiency of magnetic refrigeration process [9, 35].
Figure 4. Temperature dependent ΔTad versus T (K) data in the GNMO sample (inset for GCMO) for different magnetic fields.
Download figure:
Standard image High-resolution image4. Conclusions
In summary, we have prepared GNMO and GCMO double perovskites by simple solid-state reaction method and studied their magnetocaloric properties. Magnetic and heat capacity measurements on GNMO sample has revealed a superior magnetocaloric performance of −ΔSM ~ 35.5 J g−1 K−1 and ΔTad ~ 10.5 K at low temperatures compared to GCMO where −ΔSM ~ 24 JKg−1 K−1 and ΔTad ~ 6.5 K. Presence of 3d–4f interactions reduce the resultant magnetocaloric effect in GCMO. Further, simple synthesis, high chemical stability, absence of magnetic and thermal hysteresis and insulating nature suggest them as potential magnetic refrigerants below the liquid hydrogen temperatures.
Acknowledgments
The authors acknowledge DST and IIT Kharagpur for funding VSM-SQUID magnetometer. Krishna thanks to CSIR-UGC, Delhi for SRF.