Abstract
A sensitive non-enzyme hydrogen peroxide (H2O2) sensor has been prepared based on Fe3O4/reduced graphene oxide (Fe3O4/RGO) nanocomposite material. The sensitivity of Fe3O4/RGO modified electrode toward the electrochemical reduction of H2O2 is higher than that of Fe3O4 or Fe3O4/GO modified electrodes. A linear range of H2O2 concentration is obtained from 5.0 × 10−7 to 3.0 × 10−3 M with a detection limit of 1.8 × 10−7 M (S/N=3). The Fe3O4/RGO modified electrode also exhibits high selectivity and stability in the detection of H2O2, which suggests that Fe3O4/RGO nanocomposite material provides a promising platform for the non-enzyme electrochemical sensor.
Export citation and abstract BibTeX RIS

This is an open access article distributed under the terms of the Creative Commons Attribution Non-Commercial No Derivatives 4.0 License (CC BY-NC-ND, http://creativecommons.org/licenses/by-nc-nd/4.0/), which permits non-commercial reuse, distribution, and reproduction in any medium, provided the original work is not changed in any way and is properly cited. For permission for commercial reuse, please email: oa@electrochem.org.
Hydrogen peroxide (H2O2) is one of the most important species involved in environmental and biological processes. The determination of H2O2 is of great importance in environmental sciences, biochemistry, food inspection, clinical analysis and other areas.1Enzyme-based electrochemical sensors have been developed for monitoring H2O2 due to their high sensitivity and selectivity.2 However, enzymes are sensitive to the environmental conditions and the instability of enzyme-based biosensors is inevitable.3 Therefore, more and more attentions have been paid on the non-enzyme electrochemical sensors with high efficiency.4,5
A variety of other types of electrodes have been used for H2O2 detection, including conductive polymers,6,7 noble metals (Pt, Pd, Ru)8–10 and metal oxides (indium-tin oxide, Ir oxide).11,12 Among them, metal oxides exhibit stable and sensitive electrocatalytic response to H2O2 and are not prone to interferences. And the main adavantage in using iron (II/III) oxide comes from the detection in electroreduction of H2O2 at less "active potentials", which shows excellent anti-interference ability.
Fe3O4 magnetic nanoparticles (NPs) have been widely used in immobilizing enzyme as inert support due to their advantages of superparamagnetism, low cost and eco-friendliness.13 In 2007, Yan et al.14 first reported that Fe3O4 NPs possess enzyme mimetic activity. This result suggests that the electrodes modified with Fe3O4-based nanoparticles might display response to H2O2. Following this idea, Fe3O4-based non-enzyme electrochemical biosensors were prepared by layer-by-layer assembly of Fe3O4 NPs and polyelectrolyte PDDA on electrode and showed electrocatalytic activity toward the reduction of H2O2.15 However, in this method, polyelectrolyte hinders electron transfer between Fe3O4 NPs and electrode, which limits the electrocatalytic activity of Fe3O4. Furthermore, Fe3O4 NPs are also prone to aggregate and result in poor magnetism and dispersity due to their large ratio of surface area to volume and strong magnetic dipole-dipole attractions between nanoparticles.16 In order to maintain the catalytic activity of Fe3O4, it is necessary to modify its surface or provide it a support.
Recently, graphene or reduced graphene oxide (RGO) has been widely used in electrochemical biosensors, especially as a support for immobilization of enzymes and noble metal nanoparticles due to its high specific surface area, excellent conductivity and good biocompatibility.17 The sensitivity of the biosensors can be greatly enhanced for that graphene provides a favorable microenvironment for enzyme and promote the direct electron transfer of enzyme at the modified electrode. Graphene sheets have every atom in their structure exposed on their surfaces, so even small changes in the charge environment caused by the adsorption of biomolecules can give measurable changes in their electrical properties.18 This structured advantage of graphene provides greater opportunity for researchers to fabricate more sensitive sensors.
Considering the advantages of both Fe3O4 NPs and RGO in biosensor, it is an urgent demand to combine these two interesting materials to modify electrode and construct H2O2 biosensor with excellent performance. Fe3O4 and RGO have been used as platform for the immobilization of NADH,19 hemoglobin,20 and iron (II) phthalocyanine21 for biosensoring H2O2 and other small molecules. However, no research work on the direct electrochemistry of Fe3O4 on RGO support is reported. In this work, we prepare a non-enzyme H2O2 biosensor based on the direct electrochemistry of Fe3O4 on RGO support. The as-prepared biosensor displays a sensitive and stable response to the reduction of H2O2, which indicates that the introduction of RGO could enhance the catalytic activity of Fe3O4 NPs toward the reduction of H2O2.
Experimental
Fe3O4 NPs are synthesized by a coprecipitation procedure as follows. FeCl3·6H2O (0.54 g, 0.02 mol) and FeCl2·4H2O (0.2 g, 0.01mol) are dissolved in 50 mL distilled water. Then 5 mL ammonia solution (25%-28%) is added under vigorous stirring. After stirring for another 2 h, the black Fe3O4 NPs are separated and washed with distilled water for 3 times until the supernatant liquid is absolutely clear. Then the as-prepared Fe3O4 NPs are dried at 60°C in a vacuum oven.
Fe3O4/GO nanocomposite materials are prepared as follows. First GO is prepared by oxidation of graphite flakes according to the method described by Hummers.22 Then FeCl3·6H2O (0.54 g, 0.02 mol) and FeCl2·4H2O (0.20 g, 0.01mol) are dissolved in 50 mL GO aqueous solution (0.01 g/mL). Then 5 mL ammonia solution (25%-28%) is added under vigorous stirring. After stirring for another 2 h, the Fe3O4/GO nanocomposite materials are separated by an external magnet and washed with distilled water for 3 times until the supernatant liquid is absolutely clear. Then the nanocomposites are dried at 60°C in a vacuum oven.
The Fe3O4 modified electrode is prepared by dropping 10 μL Fe3O4 aqueous solution (0.5 mg/mL) onto the surface of glassy carbon electrode (GCE) and drying at room temperature (RT). Then 5 μL 0.05% Nafion solution is casted onto the GCE surface to fasten the Fe3O4 NPs. After drying, the Fe3O4 modified biosensor is stored in ambient atmosphere at RT for later electrochemical measurements.
The Fe3O4/GO modified electrode is prepared using the same method. 10 μL Fe3O4/GO aqueous solution (0.5 mg/mL) is dropped onto the GCE surface and dried at RT. Then 5 μL 0.05% Nafion solution is casted onto the GCE surface. After drying, the Fe3O4/GO modified biosensor is stored in ambient atmosphere at RT for later electrochemical measurements.
The Fe3O4/RGO modified electrode is prepared by direct electrochemical reduction of Fe3O4/GO modified electrode. Mainly, the Fe3O4/GO modified electrode is scanned from -0.6 to -1.0 V for 100 cycles in 0.1 M PBS solution containing 0.5 M KCl (pH 7.0). The as-prepared Fe3O4/RGO modified electrode is rinsed with distilled water for 3 times and then stored in ambient atmosphere at RT for later electrochemical measurements.
Results and Discussion
Characteristics of the Fe3O4/GO nanocomposite material
The XRD pattern of Fe3O4/GO and Fe3O4/RGO nanocomposite materials are shown in Fig. 1A. In the XRD pattern of Fe3O4/GO, the distinct diffraction peaks at 2θ = 17.9, 30.2, 35.6, 43.3, 53.7, 57.3 and 62.8 are indexed as the (111), (220), (311), (400), (422), (511), and (440) facets of cubic Fe3O4 (JCPDS No. 89–4319). In the XRD pattern of Fe3O4/RGO, the peaks of Fe3O4 are remained, showing that no Fe3O4 is reduced by the electrochemical reduction. The main peaks of Fe3O4 in the XRD pattern are broadened, indicating the Fe3O4 particles are very small. The crystalline size of Fe3O4 particles calculated from Scherrer formular is ca. 30 nm, which is in accordance with the SEM image (Fig. 1B).
Figure 1. XRD pattern (A) and SEM image (B) of Fe3O4/RGO nanocomposite material.
Fig. 2 shows the FTIR spectra of GO, RGO, Fe3O4/GO and Fe3O4/RGO. In the FTIR spectrum of GO, the band at 3200 cm−1 is attributed to the O-H streching vibration and the band at 1725 cm−1 is attributed to C=O streching vibration. The band at 1620 cm−1 is attrituted to C=C streching vibration and the band at 1398 cm−1 is attributed to C-OH stretching vibration. In the FTIR spectrum of RGO, the band at 3200 cm−1 of O-H streching vibration and the band at 1725 cm−1 of C=O streching vibration disappear, showing that GO is reduced to RGO. In the FTIR spectrum of Fe3O4/GO, the broad band at 600 cm−1 is attributed to Fe-O vibration of Fe3O4. The C=O streching vibration band of GO at 1725 cm−1 disappears and the bands at 3200 and 1575 cm−1 of O-H streching vibration and bending vibration appear, indicating that C=O group is partially reduced to C-OH group. In the FTIR spectrum of Fe3O4/RGO, the C=C streching vibration at 1620 cm−1 is observed, indicating that GO is totally elecro-reduced to RGO. The Fe-O vibration band at 600 cm−1 indicates the presence of Fe3O4 after Fe3O4/GO is electroreduced to Fe3O4/RGO.
Figure 2. FT-IR spectra of GO, RGO, Fe3O4/GO and Fe3O4/RGO.
Electrochemical studies of the biosensors
Electrochemical impedance spectroscopy (EIS) is used to evaluate the electron transfer resistance (Ret) of the modified electrodes. Fig. 3 shows the Nyquist plots of the impedance spectroscopy of the bare GCE, Fe3O4, Fe3O4/GO and Fe3O4/RGO modified electrodes. The Ret values are 20, 5500, 210, 120 Ω, respectively. Compared with the bare GCE, the non-conducting Fe3O4 coated on the electrode blocks the electron transfer of the redox probe [Fe(CN)6]3-/4− to the electrode surface, resulting in an obviously increase of Ret. When Fe3O4 is embedded on the surface of GO, the Ret of Fe3O4/GO decreases from 5500 to 210 Ω, indicating that equivalent quantity of Fe3O4 on GO can facilitate the electron transfer of the redox probe [Fe(CN)6]3-/4− to the electrode surface. When Fe3O4/GO modified electrode is electrochemically reduced, the Ret of Fe3O4/RGO modified electrode is further decreased to 120 Ω, indicating that RGO is more conductive. The improvement of the interfacial conductivity on Fe3O4/RGO modified electrode can be attributed to the excellent electrical conductivity of RGO, which can promote the electron transfer process.
Figure 3. EIS response of bare GCE (a), Fe3O4 modified electrode (b), Fe3O4/GO modified electrode (c), and Fe3O4/RGO (d) modified electrode in 10 mM K3Fe(CN)6/K4Fe(CN)6 solution containing 0.2 M KCl. Amplitude: 5 mV, frequency range: 1∼100000 Hz.
Fig. 4 compares the cyclic voltammograms of Fe3O4, Fe3O4/GO, and Fe3O4/RGO modified electrodes in PBS solution with and without hydrogen peroxide. The CV peaks of Fe3O4 in the blank solution (dashed lines) increase from Fe3O4 to Fe3O4/GO and then to Fe3O4/RGO. It indicates that GO and RGO support increase the sensitivity of Fe3O4. The electrode loaded with Fe3O4/GO nanocomposites exhibits higher catalytic current than Fe3O4 for the electrochemical reduction of H2O2. And when the Fe3O4/GO is further electroreduced to Fe3O4/RGO, significant improvement of the catalytic current is observed for the electrochemical reduction of H2O2. The improvement of Fe3O4/RGO modified electrode can be attributed to the excellent conductivity of RGO.
Figure 4. Cyclic voltammograms of graphite electrode (A), Fe3O4 (B), Fe3O4/GO (C) and Fe3O4/RGO (D) modified electrodes in 0.1 M PBS solution (pH 7.0) with (solid line) and without (dashed line) 5 mM H2O2. Scan rate: 20 mV·s−1.
From Fig. 4, it can be seen that there are a couple of redox peaks on the Fe3O4 catalysts. When H2O2 is added, the cathodic peak current is increased and the anodic peak current is decreased. So the mechanism of the reduction of H2O2 on the Fe3O4 catalysts can be proposed in Scheme 1. The reaction is composed of an electron transfer process between Fe3+ and Fe2+ on the Fe3O4 catalysts followed by a chemical reduction reaction of H2O2 by Fe2+ ("EC'" mechanism).23
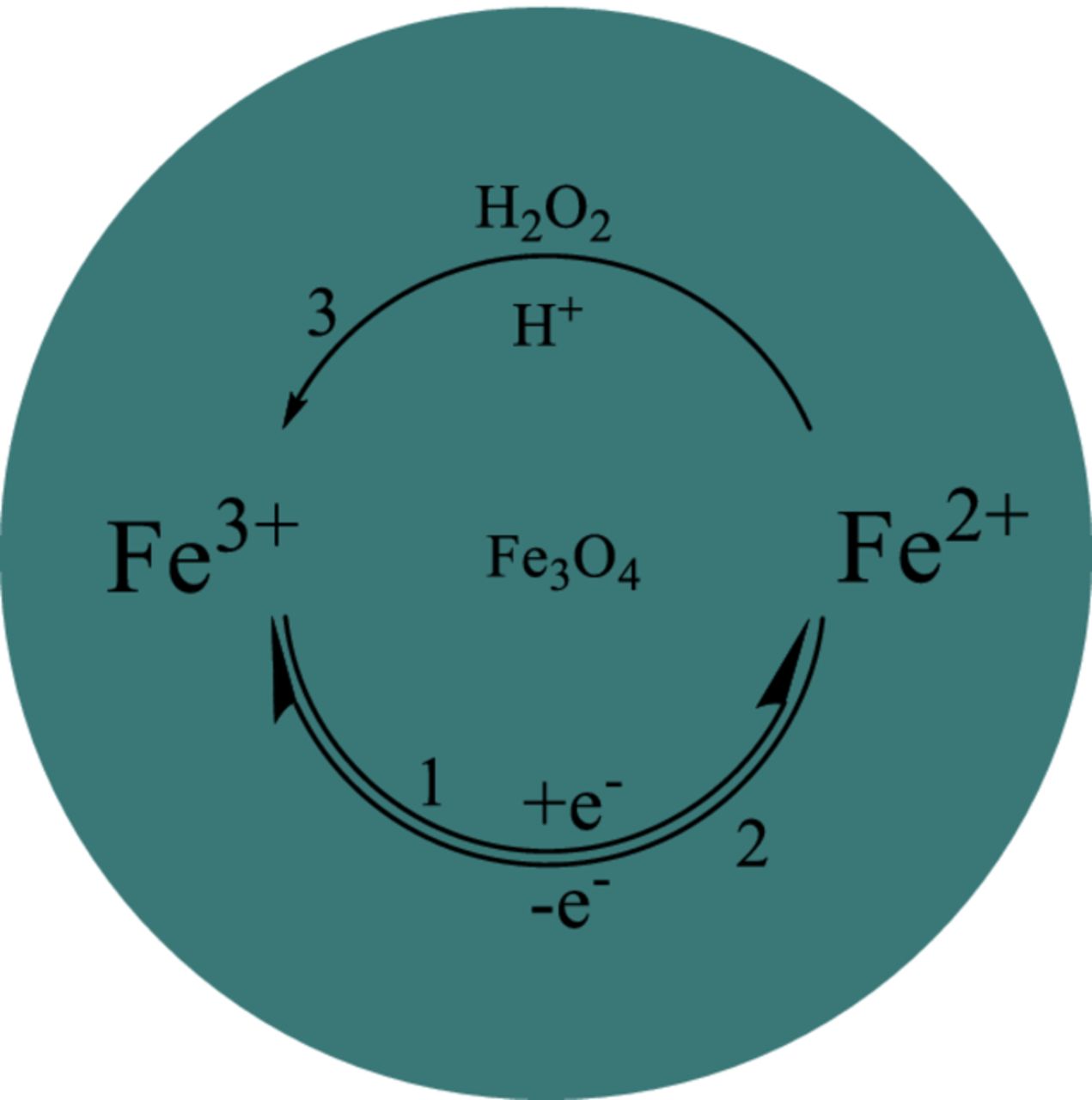
Scheme 1. Proposed mechanism of the catalytic reduction of H2O2 on Fe3O4 catalysts.
Dectection of H2O2 on the Fe3O4 modified electrodes
The current responses for the successive injection of H2O2 on the modified electrodes are also studied. The typical i-t curves are presented in Fig. 5. The sensitivity of Fe3O4 modified electrode is 2.30 μA·mM−1·cm−2, and the linear range is from 2.0 × 10−4 to 3.0 × 10−3 M and the detection limit is 1.1 × 10−4 M (S/N=3). The sensitivity of Fe3O4/GO modified electrode is increased to 11.23 μA·mM−1·cm−2, and the linear range is from 2.0 × 10−5 to 3.0 × 10−3 M and the detection limit is 1.3 × 10−5 M (S/N=3). When the Fe3O4/GO is electroreduced to Fe3O4/RGO, the sensitivity of Fe3O4/RGO modified electrode is further increased to 22.27 μA·mM−1·cm−2. The linear relationship between the current response and H2O2 concentration is over the range of 5.0 × 10−7 M to 3.0 × 10−3 M (R=0.9966) and the detection limit is as low as 1.8 × 10−7 M (S/N=3).
Figure 5. (A) Typical steady-state current responses of Fe3O4 (a), Fe3O4/GO (b), and Fe3O4/RGO (c) modified electrodes to the successive additions of H2O2 in 0.1 M PBS solution (pH 7.0). Potential applied: -0.3 V. (B) Calibration curves of the current response on the H2O2 concentration.
The interference and stability of Fe3O4/RGO modified electrode
The effects of foreign interference species on the determination of H2O2 at Fe3O4/RGO modified electrode are investigated and presented in Fig. 6. It is found that 10-fold of reductive interferences, such as L-cysteine, ascorbic acid, uric acid and glucose, do not affect the determination of H2O2. However, oxidative interferences, such as MnO4−, Cr2O72−, and Fe3+, affect the measurement of H2O2 at the applied potential of -0.3 V. Therefore, excellent interference resistibility of Fe3O4/RGO modified electrode can be achieved at reductive potential of -0.3 V for reductive interference species.
Figure 6. The amperometric H2O2 response of Fe3O4/RGO modified electrode in the presence of different interferences.
The reproducibility of the Fe3O4/RGO modified electrode is investigated by amperometric measurement of 20 μM H2O2 in 0.10 M PBS solution (pH 7.0). Six electrodes are fabricated based on the same construction process to examine their amperometric responses, and the relative standard derivation (RSD) is 5.4%. The repeatability of one electrode is also examined by six successive measurements, and the RSD is 2.8%. The Fe3O4/RGO modified electrode exhibits high reproducibility and repeatability.
The stability of the Fe3O4/RGO modified electrode is investigated by storing it in ambient atmosphere at RT and measuring once a day over a period of 30 days. The current response remains 91% of its initial value after one month, indicating high stability of the non-enzyme H2O2 biosensor.
Conclusions
In summary, a non-enzyme H2O2 sensor based on Fe3O4/RGO nanocomposite material is prepared. The Fe3O4/RGO modified electrode exhibits higher sensitivity toward the electrochemical reduction of H2O2 than Fe3O4 or Fe3O4/GO modified electrodes. A linear range of H2O2 concentration is obtained from 5.0 × 10−7 to 3.0 × 10−3 M with a detection limit of 1.8 × 10−7 M (S/N=3). The Fe3O4/RGO modified electrode also shows high selectivity, repeatability and stability in the detection of H2O2. It suggests that Fe3O4/RGO nanocomposite material provides a promising platform for the non-enzyme electrochemical sensor.
Acknowledgment
This work is financially supported by National Natural Science Foundation of China (21163007, 21165009, 21465011), Project of Jiangxi Academic and Technological leader (20133BCB22007), and Natural Sciene Foundation of Jiangxi Province (20132BAB203012).