Abstract
(
, Pr, Nd, Sm, and Gd) perovskite oxides have been synthesized and compared as cathode materials for intermediate temperature solid oxide fuel cells (SOFC). Both the electrical conductivity and the oxide ion vacancy concentration decrease from
to Gd, which leads to a decrease in the electrocatalytic activity for oxygen reduction in SOFC. However, the thermal expansion coefficient (TEC) decreases favorably from
to Gd due to a decreasing ionicity of the Ln–O bond and a suppression of the tendency to lose oxygen from the lattice with increasing temperature. Therefore,
cathodes with an intermediate lanthanide ion such as
or
offer a trade-off between electrocatalytic activity and TEC. Also, the differences in dependence of the properties of the
and
systems on the
ions are discussed.
Export citation and abstract BibTeX RIS
The chemical reactivity and thermal expansion mismatch among the components as well as the limited choice of interconnect and cathode materials at the conventional operating temperatures of solid oxide fuel cells (SOFC) have created enormous interest recently in intermediate temperature
SOFC.1 However, the performance of the intermediate temperature SOFC is strongly dependent on the electrochemical properties of the cathode materials because the cathode overpotential for the oxygen reduction reaction (ORR) increases significantly at lower temperatures.2 Therefore, there is a need to develop alternate mixed ionic-electronic conductors that can replace the conventional
cathodes.
A desirable cathode material for the intermediate temperature SOFC should have high electronic and oxide ion conductivities, chemical and thermal expansion compatibility with the electrolyte, and high catalytic activity for the oxygen reduction reaction. In this regard, the Sr-doped lanthanum cobaltites have been investigated intensively due to their high electronic and oxide ion conductivities.3, 4 However, the
cathodes exhibit a large thermal expansion coefficient (TEC) and react readily with the yttria-stabilized zirconia (YSZ) electrolyte.5, 6 Accordingly, other Sr-doped lanthanide cobaltites
have also been investigated as cathode materials for intermediate temperature SOFC.7–12 Despite a scattered investigation of the
cathodes, a systematic investigation of the factors that control their electrocatalytic activity toward oxygen reduction reaction is scarcely available in the literature. With this perspective, we present here a comparison of the crystal chemistry, thermal expansion, electrical conductivity, chemical compatibility, and electrochemical performance of the
(
, Pr, Nd, Sm, and Gd) cathodes and a correlation of the electrochemical performance to the lanthanide host cations. Additionally, the differences in the influence of the
ions on the properties of the
and
systems are discussed.
Experimental
The (
, Pr, Nd, Sm, and Gd) cathode samples were synthesized by conventional solid-state reaction method. Required amounts of the lanthanide oxides (
,
,
,
, or
),
, and
were thoroughly mixed with ethanol using an agate mortar and pestle, and calcined in air first at
for
. The calcined powders were then ground, pressed into pellets, and finally sintered at
for
. The sintering at
was repeated again for
after regrinding and repelletizing to improve the product homogeneity. The
(LSGM) electrolyte disks were prepared by firing required amounts of
,
,
, and MgO at
for
, followed by pelletizing and sintering at
for
.
(GDC) cermet
anode was synthesized by the glycine-nitrate combustion method.13
The products thus obtained were characterized by X-ray diffraction (XRD) and the lattice parameters were obtained by analyzing the XRD data with the Rietveld method. Brunauer, Emmett, and Teller (BET) surface area was measured with a Quantachrom Autosorb-1 surface area and pore size analyzer. The average oxidation state of Co and the oxygen content at room temperature were determined by iodometric titration14 with fine powders obtained by ballmilling the oxides and sieving them a few times; the fine powder was necessary to have a quantitative dissolution of the oxides in a mixture of 10% KI and HCl solution during iodometric titration. Thermogravimetric analysis (TGA) and thermal expansion data were collected with a Perkin-Elmer series 7 thermal analysis system. The TGA experiments were carried out from room temperature to
with a heating rate of
and a cooling rate of
in air. The TECs of sintered samples were measured from room temperature to
with a heating/cooling rate of
. Electrical conductivity of the samples was measured by a four-probe dc method in the temperature range of
. The bulk densities of the sintered samples were measured by the Archimedes method. All the samples used for thermal expansion and conductivity measurements had densities of
of theoretical values. The specimens for the reactivity tests were obtained by mixing the cathode powders with the LSGM electrolyte in a 1:1 weight ratio. The reactivity tests were then carried out at
for
in air. The microstructures of the cathodes were studied with a JEOL LSM-5610 scanning electron microscope.
measurements of single cells were carried out using a three-electrode configuration which allowed for separating and monitoring the cathode overpotentials during operation. Pt paste was used as the reference electrode. The
cathodes and NiO-GDC cermet anode were prepared by screen printing onto
thick LSGM electrolyte pellet, followed by firing for
at
for the cathode and
for the anode. The geometrical area of the electrode was
. Humidified
(
at
) and air were supplied as fuel and oxidant, respectively, at a rate of
.
Results and Discussion
X-ray diffraction showed all the samples to be single-phase perovskite solid solutions without any detectable impurity phases, which is consistent with the report of Ohbayashi et al.12 While the
sample was found to be rhombohedral, all others with
, Nd, Sm, and Gd were found to be orthorhombic. The lattice parameters, lattice volume, pseudo-cubic lattice parameter, and tolerance factor
for all the
samples are summarized in Table I. The pseudo-cubic lattice parameter decreases from
to Gd due to a decrease in the ionic radius of the
ion. The Goldschmidt tolerance factor
can be used as a measure of the deviation of the
perovskite structure from the ideal cubic symmetry,
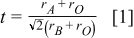
where ,
, and
are, respectively, the radii of
,
, and
ions. A decreasing
due to a decreasing ionic radius from
to
causes a lowering of the crystal symmetry and an increasing bending of the O–Co–O bond angle from the ideal value of 180°.
Table I. Lattice parameters, lattice volume, pseudocubic lattice parameter , tolerance factor
, and chemical analysis data of
.
Ln |
![]() |
![]() |
![]() | Lattice volume ![]() |
![]() |
![]() | Oxidation state of Co | Oxygen content![]() |
---|---|---|---|---|---|---|---|---|
La | 5.4079(4) | - | - | 112.5 | 4.8274 | 0.965 | 3.38 | 2.99 |
Pr | 5.3733(5) | 5.4240(5) | 7.5962(8) | 221.4 | 3.8110 | 0.957 | 3.39 | 3.00 |
Nd | 5.3656(5) | 5.4148(4) | 7.5917(7) | 220.6 | 3.8064 | 0.953 | 3.38 | 2.99 |
Sm | 5.3564(6) | 5.3814(5) | 7.5792(8) | 218.5 | 3.7943 | 0.946 | 3.39 | 3.00 |
Gd | 5.3578(9) | 5.3654(4) | 7.5718(8) | 217.7 | 3.7897 | 0.941 | 3.40 | 3.00 |
The average oxidation state of Co and the oxygen content values determined at room temperature by the iodometric titration are given in Table I for the oxides. The oxygen contents remains at 3.0 irrespective of the lanthanide ion. The TGA plots comparing the variations of oxygen contents in
with temperature are shown in Fig. 1. The degree of oxygen loss decreases from
to Gd, suggesting a stronger binding of the oxide ions to the lattice. This is consistent with a report that the enthalpies of formation of solid oxides
and their lattice energies increase from
to Gd.15 The TGA data imply that the tendency for oxygen nonstoichiometry (vacancy concentration) in
(
, Pr, Nd, Sm, and Gd) decreases in the order
.
Figure 1. Comparison of the TGA plots of (
, Pr, Nd, Sm, and Gd) recorded in air with a heating rate of
.
The temperature dependence of the electrical conductivity of (
, Pr, Nd, Sm, and Gd) is shown in Fig. 2. All the samples exhibit metallic conduction and have conductivity values of
at
. The faster decrease in conductivity at higher temperatures could be due to the formation of significant amount of oxide ion vacancies as indicated by the TGA data (Fig. 1). The formation of oxide ion vacancies is accompanied by a reduction of
to
, resulting in a decrease in the charge carrier concentration and Co–O covalency. Also, the oxide ion vacancies will perturb the O–Co–O periodic potential and covalent interaction.16 These factors lead to a decrease in electrical conductivity at elevated temperatures.
Figure 2. Comparison of the temperature dependence of the electrical conductivity of (
, Pr, Nd, Sm, and Gd) in air.
At a given temperature, the electrical conductivity of the (
, Pr, Nd, Sm, and Gd) samples decreases from
to Gd. This can be understood by considering the changes in the structural parameters. As seen in Table I, the decreasing tolerance factor
with decreasing ionic radius from
to
increases the bending of the O–Co–O bonds (lowers the O–Co–O bond angle from 180°), which results in a decrease in the overlap between the
and
orbitals and bandwidth. Thus, the decreasing covalency of the Co–O bonds and the increasing electron localization from
to
causes a decrease in electrical conductivity.
The average thermal expansion coefficients (TECs) of the samples measured in the range of
in air are given in Table II. The TEC value decreases from
to Gd. A similar trend has been observed in the analogous
17 and
18 systems also. In general, ionic bonds have a larger thermal expansion than the covalent bonds. Therefore, the variations in TEC can be understood by considering the ionic character of the Ln–O bonds. Mori et al.19 have also discussed the variations of TEC in the analogous lanthanum manganites
(
-earth) in terms of the ionic character of the
bond. The percent ionic character of a bond is related to the electronegativity difference between the bonded atoms
and
in the
bond by the following empirical relationship:20

where and
are the electronegativities of the
and
atoms, respectively. Based on the Pauling electronegativity values,21 one can, therefore, understand that the decreasing TEC from
to Gd is due to the increasing electronegativity of Ln and the decreasing ionic character of the Ln–O bond from
to Gd.
Table II. BET surface area, average crystallite size, and average thermal expansion coefficient (TEC) of .
Ln | BET surface area![]() | Average crystallite size |
![]() ![]() |
---|---|---|---|
La | 4.6 | 510 | 21.3 |
Pr | 5.2 | 480 | 19.5 |
Nd | 4.0 | 550 | 18.7 |
Sm | 3.7 | 580 | 18.0 |
Gd | 4.8 | 500 | 17.1 |
bAverage crystallite size was estimated with the line broadening of XRD peaks, and the error bar is .
Additionally, the variations in TEC could also be related to the tendency to form oxide ion vacancies as the sample is heated. The formation of oxide ion vacancies can cause an increase in TEC due to the reduction of the smaller ions to the larger
ions.3, 22 Furthermore, TEC is inversely proportional to the binding energy between the ions in the lattice.23 Hayashi et al.24 have reported that the TEC of GDC increases with the amount of oxide ion vacancies due to a reduction in the binding energy of the metal-oxygen bonds. Therefore, the increasing Ln–O bond strength15 and the decreasing degree of oxygen loss, as indicated by the TGA data in Fig. 1, from
to Gd can be considered to cause a decrease in TEC. In addition, the larger TEC of the cobaltites is partly related to the transition of the
ions from a low spin (
,
) to high spin (
,
) state.25 A suppression of such spin-state transitions by the decreasing amount of
ions (or the decreasing amount of oxide ion vacancies) at elevated temperatures on going from
to Gd could also contribute to the decreasing TEC.
The influence of the formation of oxide ion vacancies on TEC is also supported by the fact that the decrease in the TEC value on going from to Gd in
(
, measured at
) is larger than that found with the analogous
system (
, measured at
).17 The increasing tendency to form oxide ion vacancies on going from
to
causes a faster increase (20%) in TEC in the cobaltite system, while the absence of formation of significant amount of oxygen vacancies in the manganite system for
results in a slower increase (14%) in TEC. Thus, the change in TEC on going from
to Gd in the manganite system is mainly due to the decreasing ionicity of the Ln–O bond while that in the cobaltite system is due to both the decreasing ionicity of the Ln–O bond and the differences in the formation of oxide ion vacancies. Furthermore, the larger TEC of the cobaltites
compared to that of the manganites
is due to the formation of oxide vacancies, spin-state transitions associated with
, and the relatively weaker Co–O bond compared to the Mn–O bond.
The BET surface area and the average crystallite size of the cathode powders do not vary significantly with the
ions as seen in Table II. Therefore, the influence of the geometrical morphology of the prepared powders on the electrochemical performance could be neglected in this study. Figure 3 shows the scanning electron microscopy (SEM) micrographs of the
cathodes after screen printing them onto the LSGM electrolyte followed by firing at
for
. Porous cathode layers of about
thick with homogeneous microstructure that are well adhered to the dense LSGM electrolyte are seen. In addition, X-ray powder diffraction patterns of the
and LSGM mixtures after heating at
for
show that there is no interfacial reaction between the cathode compositions and the LSGM electrolyte (Fig. 4).
Figure 3. SEM micrographs of the cathode-LSGM electrolyte assemblies after firing at
for
: (a)
; (b)
; (c)
; (d)
; and (e)
.
Figure 4. X-ray powder diffraction patterns recorded after heating the cathode and the LSGM electrolyte powders at
for
.
The variations of the cell voltage, power density, and overpotential with current density at are compared in Fig. 5 for the various
cathodes. The power density decreases and the overpotential increases from
to Gd. The cathode overpotential for oxygen reduction reaction is closely related to both the electronic and oxide ion conductivities of the cathode materials because the electrocatalytic reaction at a porous cathode is limited by the kinetics of oxygen exchange and diffusion as well as the charge transfer. Both the electrical conductivity (Fig. 2) and the concentration of oxide ion vacancies (Fig. 1) decrease from
to Gd in
; oxide ion conductivity is proportional to the amount of oxide ion vacancies. Additionally, Kharton et al.26 have reported that a decrease in the radius of the
ions in
causes a decrease in the size of the anion transfer channel and an increase in the (Ln,Sr)–O bond energy, as evident from the decreasing cell volume in Table I. This suggests that the oxide ion conductivity of
would decrease in the order
, which is consistent with that found by Kharton et al.26 for
, Pr, and Nd from oxide ion conductivity measurements. Gao et al.27 have also reported that high oxide ion vacancy concentration in the surface of cathode could improve the dissociation of oxygen molecule
(ad) into atomic oxygen
. Thus, the decreasing electronic and oxide ion conductivities from
to Gd in
leads to a decrease in the oxygen exchange, transport speed of oxide ions, and charge transfer kinetics, which in turn results in a decrease in the electrochemical performance.
Figure 5. Electrochemical performance data of the single cells at
: variations of the (a)
curves (open symbols) and power densities (closed symbols) and (b) cathode overpotential.
However, considering the long-term performance, may encounter a faster degradation in electrochemical performance compared to
due to the larger difference in TEC (Table II) between the
cathode
and the LSGM electrolyte
,28 which could cause microcracks or delamination. Additionally, Qiu et al.29 have reported that
with smaller lanthanide ions is less reactive with the YSZ electrolyte than
. Therefore, the
cathodes with an intermediate lanthanide ion such as
may be preferred, considering the trade-off between electrochemical performance and other parameters like TEC and reactivity.
Unlike in the system, the cathode overpotential in the
system, however, does not seem to depend strongly on the lanthanide host cation.17 This could be related to the differences in the formation of oxide ion vacancies and oxide ion conductivity. Wen et al.30 have reported that the
concentration increases proportionately with the
concentration in the
system and oxide ion vacancies do not exist even at high temperatures. Because the
system17 does not contain oxide ion vacancies, the change in lanthanide host cations may not significantly affect the oxygen exchange kinetics, and the oxygen reduction reaction could be rather limited by the three-phase boundary or surface diffusion, resulting in no strong correlation between the lanthanide host cations and the electrochemical performance.
Conclusions
The electrocatalytic activity of (
, Pr, Nd, Sm, and Gd) for oxygen reduction reaction in SOFC decreases from
to Gd. This trend could be understood to be due to the decreasing electrical and oxide ion conductivities caused, respectively, by an increasing bending of the O–Co–O bonds and a decreasing oxide ion vacancy concentration. However, going from
to Gd provides an important advantage of a decrease in TEC, which could be understood to be due to the decreasing ionicity of the Ln–O bonds, the suppression of the reduction of the
ions to
ions, and the decreasing amount of oxide ion vacancies at elevated temperatures. These two opposing factors with decreasing
size may make the
cathodes with an intermediate lanthanide ion more attractive for practical cells. Additionally, the lack of a strong dependence of the catalytic activity of the analogous
system on the
ions unlike in the cobaltite system could be understood by considering the absence of oxide ion vacancies for
.
Acknowledgment
This work was supported by the Welch Foundation grant F-1254 .
University of Texas at Austin assisted in meeting the publication costs of this article.