Ag Loading Enhanced Photocatalytic Activity of g-C3N4 Porous Nanosheets for Decomposition of Organic Pollutants
- 1Institute of Catalysis for Energy and Environment, College of Chemistry and Chemical Engineering, Shenyang Normal University, Shenyang, China
- 2Key Laboratory of Advanced Energy Materials Chemistry (Ministry of Education), Nankai University, Tianjin, China
- 3Department of pharmacology, Shenyang Medical College, Shenyang, China
- 4Department of Hydrogen Energy, Faculty of Energy and Fuels, AGH University of Science and Technology, Kraków, Poland
- 5School of Material Science and Technology, Jilin Institute of Chemical Technology, Jilin City, China
The g-C3N4 porous nanosheets with different loading amount of Ag nanoparticles (NPs) are successfully prepared by a simple liquid-phase reduction method. These Ag/g-C3N4 composites have an improved photocatalytic performance for decomposing organic pollutants compared with that of pure g-C3N4 nanosheets. Many measurements have been used for characterizing the samples, such as XRD, FTIR, UV-Vis DRS, PL, XPS, EDS, SEM, and TEM. In Ag/g-C3N4, the Ag NPs are uniformly coated on the g-C3N4 surface, the diameter is mainly in the range of 8~18 nanometers. Loading of Ag NPs expand the response to the visible light for g-C3N4 and increasing the producing rate of photogenerated e−-h+ pairs. The loading of silver NPs obviously enhances the photocatalytic activity of C3N4 nanosheets toward the Rhodamine B (RhB) decomposition under the simulated sunlight irradiation. With different loading amounts of Ag NPs, Ag/g-C3N4 (3 wt% of Ag) showed the highest photocatalytic activity for RhB decomposition among these as-prepared samples, which is 10 times of the rate of pure C3N4. Based on the experimental results, a possible photocatalytic mechanism for Ag/g-C3N4 is proposed.
Introduction
The organic pollutants in waste water has become a serious problem that threatens human health (Zhang and Wen, 2008). Solar energy, as a clean energy, has been widely concerned. It is a very effective way to solve the problem of water pollution by using solar energy (George et al., 2015; Wang et al., 2017, 2018a; Qi et al., 2018a; Shi et al., 2018; Wei et al., 2018; Yan et al., 2018a,b; Zhong et al., 2018). Since Fujishima et al. reported the phenomenon of water splitting for hydrogen production on TiO2 photoanode (Fujishima and Honda, 1972), the study of semiconductor photocatalysts has become very popular (Tao et al., 2014; Wei et al., 2015; Wen et al., 2015; Park et al., 2016; Qi et al., 2017b, 2018b; Xia et al., 2017).
The photocatalysis technology has been widely used to treat the organic polluted waste water because of its simpler equipment, convenient operation, energy saving, environmental protection, and strong oxidizing ability. Semiconductor photocatalysts mainly include graphite nitride (g-C3N4) (Cao et al., 2015), metal oxide (Qi et al., 2017a), and metal sulfide (Hong et al., 2015). Among these materials, g-C3N4, as an important photocatalyst, has received widely attention, because of its unique characteristics, including metal free, non-toxic, easy preparation, suitable band gap, and cheap (Cao and Yu, 2014). However, the photocatalytic activity of g-C3N4 is still very low, it is difficult to use in real life, due to the low utilization efficiency of sunlight and the fast recombination of photogenerated e−-h+ pairs (Martha et al., 2013; Zhu et al., 2018). Up to now, many efforts have been devoted to improve the photocatalytic performance of g-C3N4 (Xiang et al., 2011; Akple et al., 2015; Xu et al., 2018; Fu et al., 2019). For example, Liu et al. used metal doping to enhance the visible light adsorption of g-C3N4 and found that it enhanced the photocatalytic activity in the photocatalysis of water splitting for hydrogen production (Niu et al., 2012). Cheng et al. demonstrated that through building heterojunction of ZnO/g-C3N4, the photocatalytic activity for the decomposition of organic dyes is enhanced (Cheng et al., 2013). Fina et al. reported that loading Pt nanoparticles (NPs) can enhance activity of g-C3N4 photocatalytic water splitting into H2 production (Fina et al., 2015). In these methods, depositing noble metals (Au, Ag, Pt, or Pd) on the g-C3N4 surface is useful to enhance the photocatalytic activity of g-C3N4 (Wen et al., 2017; Tong et al., 2018; Wang et al., 2018b). However, the mechanism of interaction between the loading noble metals and C3N4, and how they work to enhance the photocatalytic activity of g-C3N4 are limited to know.
This work reports a simple liquid-phase reduction method to prepare the Ag/g-C3N4 composites. The structure, morphology, optical property, and photocatalytic activity of the as-prepared Ag/g-C3N4 samples are investigated. The effects of loading content of Ag on the light absorbency and photocatalytic activity of C3N4 are studied. Under the simulated sunlight, the photocatalytic performance of g-C3N4 for Rhodamine B (RhB) photodegradation is obviously improved after loading Ag NPs. Finally, a possible photocatalytic mechanism of the Ag/g-C3N4 composite is given.
Experimental
Synthesis
The g-C3N4 nanosheet was prepared via thermal polycondensation of urea. Fifteen grams urea was placed into a covered ceramic crucible and heated to 500°C for 5 h in air, at the heating rate of 10°C min−1. After the reaction, it cooled down to room temperature naturally, the product was collected and grind to powder. The Ag/g-C3N4 composite was synthesized by a liquid-phase reduction method. First 0.5 g of g-C3N4 was put in 50 mL of water and ultrasonic treated for 5 min. Second, a certain amount of AgNO3 (5 mM) aqueous solution was put into the above solution and maintain stirring. Third, a certain amount of NaBH4 [the molar ratio of n(AgNO3):n(NaBH4) = 1:5] dissolved in 30 mL of water, and then put into the above solution, stirring for 1 h. Following, the product was centrifuged and washed with absolute ethanol and distilled water, respectively. Finally, these samples are dried in vacuum oven at 70°C for 5 h. By varying the amount of using AgNO3, a series of samples with different ratios of Ag to g-C3N4 [m(Ag):n(g-C3N4) = 1, 2, 3, 4, and 5%] were prepared and labeled as 1%-Ag/g-C3N4, 2%-Ag/g-C3N4, 3%-Ag/g-C3N4, 4%-Ag/g-C3N4, and 5%-Ag/g-C3N4, respectively.
Characterization
The crystal phases of products were studied by X-ray diffraction (XRD) (X-ray diffractometer, Cu Kα, λ = 1.54056 Å) (Bruker D5005, Germany). Fourier transform infrared (FT-IR) spectra were conducted using a Nicolet Magna 560 (US) spectrophotometer. X-ray photoelectron spectroscopy (XPS) was measured on a PHIQ 1,600 XPS (US) instrument. The weight percentages of Ag in the Ag/g-C3N4 photocatalysts was studied by inductively coupled plasma atomic emission spectrometry (ICP-AES, Shimadzu ICP-7510, Japan). High resolution transmission electron microscopy (HRTEM) was taken by a JEOL JEM-2100F (Japan) electron microscope. UV–vis absorbance spectra were collected on a Shimadzu UV-3100 (Japan) spectrophotometer, using BaSO4 as reference. The photoluminescence (PL) spectra of g-C3N4 and Ag/g-C3N4 samples were studied on a Varian Cary Eclipse (US) spectrometer equipped with an excitation wavelength of 325 nm.
Photocatalytic Performance
The photocatalytic activity of pure g-C3N4 and Ag/g-C3N4 samples was examined by photodegradation of RhB under the simulated sunlight irradiation, which was obtained from an 500 W Xe lamp. Ten milligram of samples were dispersed in 25 mL of RhB aqueous solution (10 mg/L RhB aqueous solution). Prior to the irradiation, the reaction solution was magnetically stirred in the dark for 30 min to get adsorption-desorption equilibrium for the dyes on photocatalyst surface. During the photocatalytic degradation, 2 mL of the sample was withdrawn from the reaction solution at the time intervals of every 15 min and then centrifuged to remove the particles. Then the concentration of RhB was examined by UV–vis spectrophotometer, at the absorbance wavelength of 553 nm. The photodegradation rate of RhB was calculated by the formula: D = C/C0 × 100%, where C0 is the initial concentration of RhB, and C is the concentration of RhB at a time t.
Photoelectrochemical Measurement
The photoelectrochemical performance was studied on a CHI 660D electrochemical work station with a standard three-electrode system. Put g-C3N4 or Ag/g-C3N4 on the ITO glass surface as the working electrode. A piece of Pt wire and a calomel electrode were used as the counter electrode and reference electrode, respectively. The electrolyte is 0.1 mol/L Na2SO4 aqueous solution. Five milligram photocatalysts were mixed with 1 mL ethanol and then the mixture was coated on 2 × 4 cm ITO glass for use as an electrode. Electrochemical impedance spectroscopy (EIS) Nyquist plots were conducted at an open current potential with an amplitude of 5 mV and the frequency range was from 105 to 1 Hz.
Results and Discussion
XRD Patterns
The crystal phase of as-prepared samples is studied by XRD measurements, and the XRD patterns are shown in Figure 1. The pure g-C3N4 nanosheets and Ag/g-C3N4 nanocomposites have two dominant peaks at 13.1° and 27.5°, indexed to g-C3N4 (JCPDS87-1526) (Yang et al., 2013b). The peak at 27.5° is ascribed to the typical (002) plane with planar distance of 0.33 nm corresponding to interlayer-stacking of aromatic segments. The peak at 13.1° with distance of 0.675 nm is indexed to the (100) plane corresponding to in-plane structural packing (Dong et al., 2011; Liu et al., 2011). Compared with the pure g-C3N4 nanosheets, the intensity of the diffraction peak at 27.5° becomes weaker with increasing content of loading Ag NPs. The diffraction peak related to Ag NPs is not found, because of the low Ag loading amount and the high dilution effect of Ag NPs on the g-C3N4 surface (Zhou et al., 2014; Fu et al., 2015). As follows, the XPS and EDS data demonstrate the existence of Ag loading on the g-C3N4 surface.
FTIR Analysis
The FTIR spectra are similar between the pure g-C3N4 nanosheets and Ag/g-C3N4 composites with different Ag loading amounts (Figure 2). The peak at 1,639 cm−1 can be ascribed to the stretching vibration of C-N groups, and the peaks at 1,242, 1,327, 1,568 and 1,408 cm−1 can be attributed to the aromatic C-N stretching vibration (Aghdam et al., 2017). The peak at 809 cm−1 corresponds to the breathing mode of triazine units (Sun et al., 2012). The peak at 3171 cm−1 is attributed to the stretching vibration of N-H group (Yang et al., 2013a). All these characteristic FTIR peaks suggest that the overall structure of g-C3N4 maintains the original form after Ag NPs loading.
TEM Images
The morphology and microstructure of the pure g-C3N4 and 3%-Ag/g-C3N4 samples were investigated by TEM measurements. The TEM image of pure g-C3N4 shows that it is a two-dimensional nanosheet with some holes in the size range of 10–30 nm (Figure 3A). TEM image of the 3%-Ag/g-C3N4 sample (Figure 3B) shows that Ag NPs, observed as black dots, uniformly disperse on g-C3N4 surfaces. The size of Ag NPs is from 6 to 20 nm, indicating that these Ag NPs are Ag clusters on the surface of g-C3N4. The size distribution of Ag NPs on 3%-Ag/g-C3N4 is presented in Figure 3C, which is mainly in the range of 8–18 nm. The energy dispersive X-ray spectrum (EDS) also confirms that Ag NPs exist on the surface of g-C3N4 (Figure 3D). Also, it shows that the 3%-Ag/g-C3N4 sample is consisted of C, N, and Ag elements, which confirms that Ag NPs successfully adsorbed on the g-C3N4 surface.
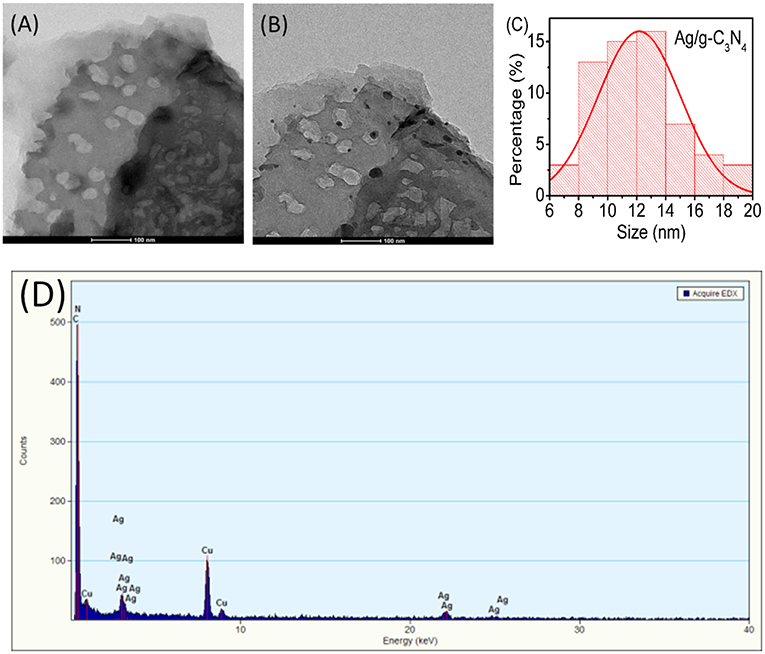
Figure 3. TEM images of (A) pure g-C3N4 and (B) 3%-Ag/g-C3N4. (C) size distribution of Ag NPs on 3%-Ag/g-C3N4. (D) EDX mapping of 3%-Ag/g-C3N4.
XPS Analysis
The surface elemental composition and chemical states of Ag/g-C3N4 are studied by XPS, here 3%-Ag/g-C3N4 is selected for study (Figure 4). The elements C, N, O, and Ag are clearly observed in the survey spectrum (Figure 4A). The peak located at 531 eV is assigned to O, which may be the water molecules at the sample surface (Hu et al., 2015). Two C 1 s peaks locate at 284.8 eV and 288.3 eV (Figure 4B). The peak located at 284.8 eV is assigned to sp2-hybridized C atoms, and the 288.3 eV peak can be assigned as N-C=N2 groups (Wu et al., 2015). As shown in Figure 4C, the peaks of N 1 s locate at 398.8, 400.5, and 401.5 eV, which can be ascribed to sp2 bonded nitrogen C-N-C groups, sp3 tertiary nitrogen N-(C)3 and amino functional groups (C-N-H), respectively (Qi et al., 2019). The spectrum of Ag 3d (Figure 4D) shows that the peaks located at 367.4 and 374.0 eV can be assigned as Ag 3d5/2 and Ag 3d3/2, respectively (Yang et al., 2016). This confirms that Ag NPs are successfully coasted on g-C3N4 surfaces. The peak at 368.1 eV is assigned as Ag(I), which indicates the formation of Ag2O on the surface of metallic Ag (Tian et al., 2015). The actual content of Ag in the Ag/g-C3N4 composites was studied by ICP-AES analysis. The result shows that the weight percentages of Ag in 1%-Ag/g-C3N4, 2%-Ag/g-C3N4, 3%-Ag/g-C3N4, 4%-Ag/g-C3N4, and 5%-Ag/g-C3N4 were measured to be 0.72, 1.43, 2.09, 2.74, and 3.55, respectively. The measured value by ICP-AES is a little smaller than the theoretical value for the weight percentages of Ag in Ag/g-C3N4 composites, but both of the two changing trends are the same.
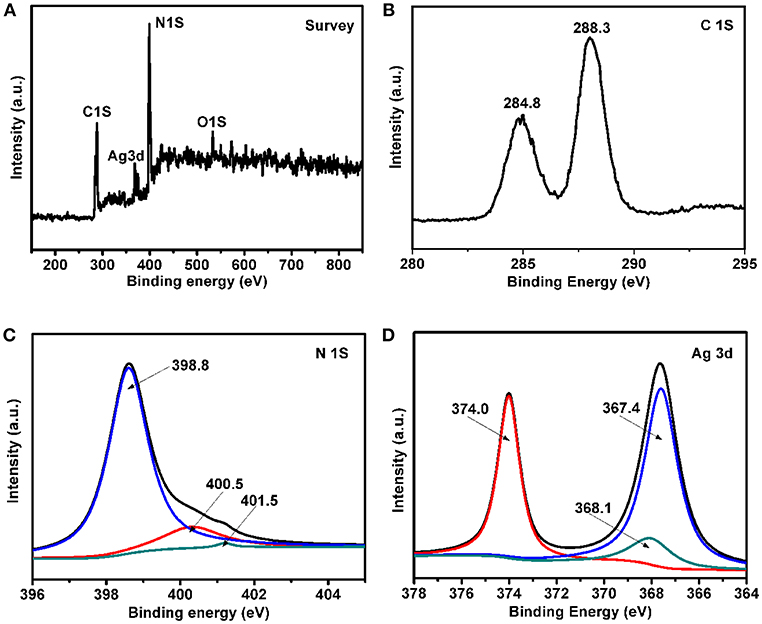
Figure 4. XPS spectra of 3%-Ag/g-C3N4 composites: (A) survey XPS spectrum, high resolution of (B) C1s spectra, (C) N1s spectrum, (D) Ag 3d spectrum.
UV-vis Diffuse Reflectance Spectra
The UV-DRS measurement is used to study the optical adsorption property of the pure g-C3N4 nanosheets and Ag/g-C3N4 composites (Figure 5). Figure 5A shows that the light absorption edge of the pure g-C3N4 is at 440 nm, which agrees with the intrinsic band gap of bulk g-C3N4 (Chen et al., 2016). Compared with pure g-C3N4 nanosheets, Ag/g-C3N4 composites have an additional weak and broad absorption peak around 450–600 nm, which is characteristic of the silver surface plasmon resonance band (Liu et al., 2013). The Ag/g-C3N4 composite shows similar light absorption range with that of pure g-C3N4, but the visible light adsorption is increased, as shown in Figure 5B. Thus, the samples with the increasing of Ag loading amount change the color from yellow to dark gray.
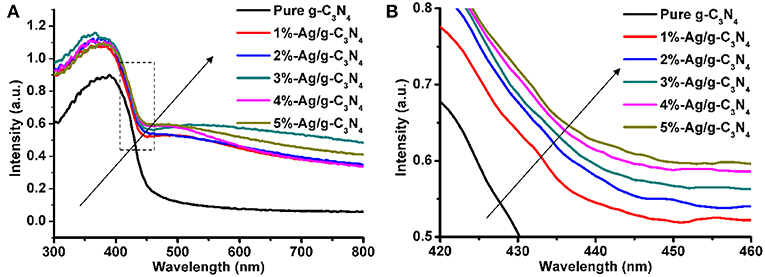
Figure 5. (A) UV-Vis diffuse reflectance absorption spectra of the pure g-C3N4 and Ag/g-C3N4 samples. (B) Magnify the square apart of picture (A).
PL Spectra
Photoluminescence measurement is an useful method to analyze the separation efficiency and the life time of photogenerated carriers, as shown in Figure 6. PL spectra of pure g-C3N4 and Ag/g-C3N4 are taken by the exciting light of 325 nm. A strong broad peak at ~460 nm is observed. Compared with pure g-C3N4, the PL intensity of Ag/g-C3N4 composites decreases significantly. The weaker peak intensity of PL results in a slower recombination rate of photogenerated carriers (Ong et al., 2014). In Ag/g-C3N4 composites, Ag NPs combine with the g-C3N4 surface strongly, and effectively reduce the recombination rate of e−-h+ pairs. This improved separation efficiency of photogenerated carriers leads to increasing e− and h+ to join the photocatalytic process of Ag/g-C3N4. However, over loading of Ag on g-C3N4, such as 5%-Ag/g-C3N4, the PL intensity is getting increase again, indicating the increase of the combination of photogenerated carriers. It clearly sees that the Ag/g-C3N4 composites with proper loading amounts of Ag have a potential for using as photocatalysts with high activity.
Photocatalytic Activity
The photocatalytic activity of pure g-C3N4 and Ag/g-C3N4 for photodecomposition of RhB is tested under the simulated sunlight irradiation. As shown in Figure 7A, compared with that of pure g-C3N4, the Ag nanoparticle modified g-C3N4 shows an improved photocatalytic performance for decomposition of RhB aqueous. After irradiation for 100 min, the degradation of RhB is about 20% for pure g-C3N4 nanosheets and almost 100% for 3%-Ag/g-C3N4. Figure 7B shows the apparent reaction rate constant (k) of RhB photodegradation, which shows that the kinetic constant of 3%-Ag/g-C3N4 is almost 10 times higher than that of pure g-C3N4. When the mass ratio of Ag is in the range of 1–5 wt%, the enhanced photocatalytic activity is observed, due to effective enhanced the separation efficiency of photogenerated e−-h+ pairs at the Ag/g-C3N4 interface and the surface plasmon resonance (SPR) effect of Ag NPs (Duan et al., 2014), which are also supported by PL and UV–vis DRS results. Obviously, 3%-Ag/g-C3N4 has the highest photocatalytic activity, which may be due to that the excess Ag NPs will be working as recombination centers, or the active site on g-C3N4 surface is blocked (Ge et al., 2011). The photocatalytic activity of as-prepared Ag/g-C3N4 composites is compared to the typical photocatalysts including TiO2 and ZnO. The photocatalytic activity of 3%-Ag/g-C3N4 (k = 0.0326 min−1) in this work is higher that TiO2 (k = 0.0084 min−1) and ZnO (k = 0.0062 min−1) reported previously (Carvalho et al., 2015; Hao et al., 2019). In order to check the reusability of as-prepared Ag/g-C3N4 photocatalysts, the recycling test was carried out. As shown in Figure 7C, the photodegradation percentage of RhB is >90% after six cycles, which indicates the as-prepared photocatalysts owns a good stability. A gradually decreased photocatalytic activity is due to the loss of photocatalyst in the recovery process.
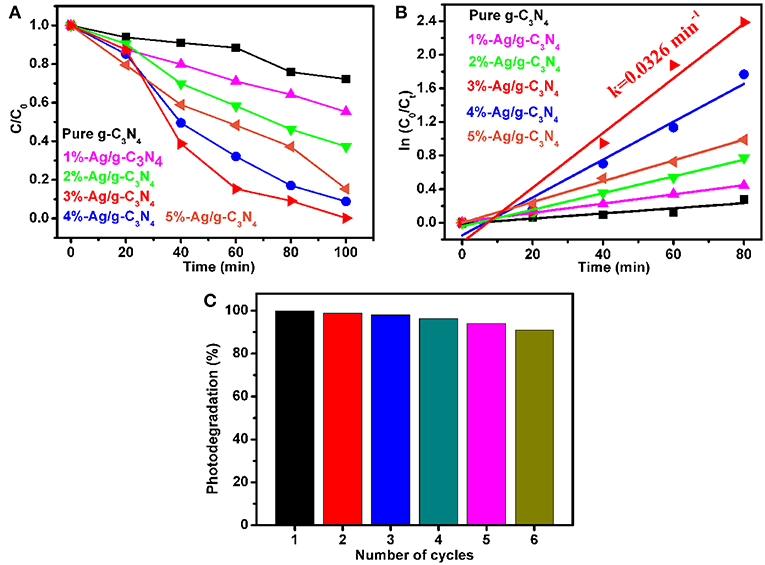
Figure 7. (A) photodegradation of RhB using pure g-C3N4, Ag/g-C3N4 with different Ag contents under simulated sunlight irradiation. (B) kinetic data for the degradation of RhB. (C) the photodegradation rate of RhB for six cycles using 3%-Ag/g-C3N4.
Photoelectrochemical Performance
The charge separation efficiency is studied by using the photoelectrochemical measurements. Figure 8A shows that the photocurrent response of pure g-C3N4, 1%-Ag/g-C3N4, 3%-Ag/g-C3N4, and 5%-Ag/g-C3N4 samples under the simulated sunlight irradiation, which shows stable reproducible photocurrent responses over five on-off cycles. The photocurrent starts when the light was turned on, the photocurrent is close to zero when the light was turned off. The photocurrent density (0.50 μA/cm2) of 3%-Ag/g-C3N4 is bigger than the pure g-C3N4 (0.07 μA/cm2) and the 5%-Ag/g-C3N4 (0.38 μA/cm2). The stronger photocurrent is due to the higher separation efficiency of the photogenerated e−-h+ pairs of Ag/g-C3N4, which is consistent with its higher activity on photocatalytic decomposition of organic dyes.
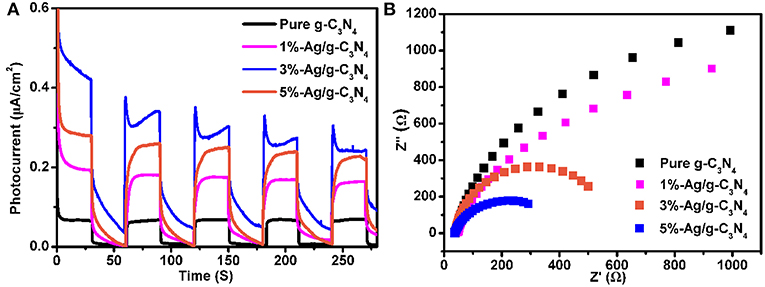
Figure 8. (A) photocurrent response and (B) electrochemical impedance spectroscopy of the pure g-C3N4, 1%-Ag/g-C3N4, 3%-Ag/g-C3N4, and 5%-Ag/g-C3N4 samples.
In order to study the charge separation efficiency, the electrochemical impedance spectroscopy (EIS) Nynquist plots are used, and the EIS Nyquist plot of pure g-C3N4, 1%-Ag/g-C3N4, 3%-Ag/g-C3N4, and 5%-Ag/g-C3N4 sample is as shown in Figure 8B. Usually, the smaller the radius of EIS Nyquist plots is, the higher the separation efficiency of charge carriers is (Li et al., 2015). The radius on the EIS Nynquist plot of C3N4 is getting smaller after Ag modification, the order is 3%-Ag/g-C3N4 < 5%-Ag/g-C3N4 < 1%-Ag/g-C3N4 < g-C3N4, indicating that Ag modification indeed reduces the recombination of charge carries and increase the separation efficiency of photogenerated e−-h+ pairs, 3%-Ag/g-C3N4 is the best, which agrees well with PL spectra.
Photocatalytic Mechanism
Figure 9 shows the photocatalytic mechanism of Ag/g-C3N4 composites during RhB decomposition under sunlight irradiation. Ag nanoparticle modification enhances the photocatalytic performance of g-C3N4 due to the synergistic effect of two aspects, one is the SPR effect of metal Ag, another is the decrease of the recombination rate of photogenerated e−-h+ pairs (Ingram et al., 2011). When Ag/g-C3N4 is irradiated by the simulated sunlight irradiation, the e−-h+ pairs are separated, e− is excited to CB of g-C3N4, h+ remains at VB of g-C3N4. Then e− transfers to Ag NPs due to the high Schottky barrier of Ag, finally, transfers to the photocatalyst surface to join the reduction reaction. The generated e− from two routes one from the plasmon excited Ag NPs and the other from the photoexcited g-C3N4 nanosheets. These e− react with O2 to generate O2−•, and O2−• radicals can discompose of RhB molecules to CO2 and H2O. Thus it is concluded that the adsorbed silver NPs have two functions, one is as the electron pool and the other is capture of the photoinduced electrons.
Conclusion
In this work, Ag NPs modified g-C3N4 nanosheets are successfully prepared by a simple liquid-phase reduction method. In the Ag/g-C3N4 composites, the Ag NPs uniformly coasted on the g-C3N4 surface with the diameter range of 6~20 nm. After Ag loading, the Ag/g-C3N4 composites expand the visible light response and show an enhanced photocatalytic activity on RhB decomposition. The enhanced photocatalytic activity of Ag/g-C3N4 is due to the two reasons, one is the SPR effect of metal Ag, and another is the decrease of the recombination of the photogenerated e−-h+ pairs. Especially, 3%-Ag/g-C3N4 demonstrates the highest photocatalytic activity among the as-prepared samples for RhB decomposition, which is 10 times faster than the pure g-C3N4 nanosheet for decomposition of RhB. This work indicates that the Ag/g-C3N4 photocatalyst is one of promising candidates to treat organic pollutants in the waste water.
Author Contributions
All authors listed have made a substantial, direct and intellectual contribution to the work, and approved it for publication.
Funding
This work was supported by National Natural Science Foundation of China (51602207 and 21801091), 111 project, Doctoral Scientific Research Foundation of Liaoning Province (20170520011), Program for Liaoning Excellent Talents in University (LR2017074), and Project of Education Office of Liaoning Province (LQN201712).
Conflict of Interest Statement
The authors declare that the research was conducted in the absence of any commercial or financial relationships that could be construed as a potential conflict of interest.
References
Aghdam, S. M., Haghighi, M., Allahyari, S., and Yosefi, L. (2017). Precipitation dispersion of various ratios of BiOI/BiOCl nanocomposite over g-C3N4 for promoted visible light nanophotocatalyst used in removal of acid orange 7 from water. J. Photochem. Photobiol. A Chem.338, 201–212. doi: 10.1016/j.jphotochem.2017.02.013
Akple, M. S., Low, J., Wageh, S., Ahmed Al-Ghamdi, A., Yu, J., and Zhang, J. (2015). Enhanced visible light photocatalytic H2-production of g-C3N4/WS2 composite heterostructures. Appl. Surf. Sci. 358, 196–203. doi: 10.1016/j.apsusc.2015.08.250
Cao, S., Low, J., Yu, J., and Jaroniec, M. (2015). Polymeric photocatalysts based on graphitic carbon nitride. Adv. Mater. 27, 2150–2176. doi: 10.1002/adma.201500033
Cao, S., and Yu, J. (2014). g-C3N4-based photocatalysts for hydrogen generation. J. Phys. Chem. Lett. 5, 2101–2107. doi: 10.1021/jz500546b
Carvalho, K. T. G., Suzane Fidelis, C., Osmando Lopes, F., and Ribeiro, C. (2015). Effect of processing variables on the photocatalytic properties of ZnO thin films prepared using the polymeric precursor method. Ceram. Int. 41, 10587–10594. doi: 10.1016/j.ceramint.2015.04.155
Chen, T., Quan, W., Yu, L., Hong, Y., Song, C., Fan, M., et al. (2016). One-step synthesis and visible-light-driven H2 production from water splitting of Ag quantum dots/g-C3N4 photocatalysts. J. Alloys Compd. 686, 628–634. doi: 10.1016/j.jallcom.2016.06.076
Cheng, N., Tian, J., Liu, Q., Ge, C., Qusti, A. H., Asiri, A. M., et al. (2013). Au-nanoparticle-loaded graphitic carbon nitride nanosheets: green photocatalytic synthesis and application toward the degradation of organic pollutants. ACS Appl. Mater. Interfaces 5, 6815–6819. doi: 10.1021/am401802r
Dong, F., Wu, L., Sun, Y., Fu, M., Wu, Z., and Lee, S. C. (2011). Efficient synthesis of polymeric g-C3N4 layered materials as novel efficient visible light driven photocatalysts. J. Mater. Chem. 21, 15171–15174. doi: 10.1039/c1jm12844b
Duan, S., Ai, Y.-J., Hu, W., and Luo, Y. (2014). Roles of Plasmonic excitation and protonation on photoreactions of p-Aminobenzenethiol on Ag nanoparticles. J. Phys. Chem. C 118, 6893–6902. doi: 10.1021/jp500728s
Fina, F., Ménard, H., and Irvine, J. T. S. (2015). The effect of Pt NPs crystallinity and distribution on the photocatalytic activity of Pt-g-C3N4. Phys. Chem. Chem. Phys. 17, 13929–13936. doi: 10.1039/C5CP00560D
Fu, J., Xu, Q., Low, J., Jiang, C., and Yu, J. (2019). Ultrathin 2D/2D WO3/g-C3N4 step-scheme H2-production photocatalyst. Appl. Catalysis B Environ. 243, 556–565. doi: 10.1016/j.apcatb.2018.11.011
Fu, Y., Huang, T., Zhang, L., Zhu, J., and Wang, X. (2015). Ag/g-C3N4 catalyst with superior catalytic performance for the degradation of dyes: a borohydride-generated superoxide radical approach. Nanoscale 7, 13723–13733. doi: 10.1039/c5nr03260a
Fujishima, A., and Honda, K. (1972). Electrochemical Photolysis of water at a semiconductor electrode. Nature 238, 37–38. doi: 10.1038/238037a0
Ge, L., Han, C., Liu, J., and Li, Y. (2011). Enhanced visible light photocatalytic activity of novel polymeric g-C3N4 loaded with Ag nanoparticles. Appl. Catalysis A Gen. 409–410, 215–222. doi: 10.1016/j.apcata.2011.10.006
George, C., Ammann, M., D'Anna, B., Donaldson, D. J., and Nizkorodov, A. (2015). Heterogeneous photochemistry in the atmosphere. Chem. Rev. 115, 4218–4258. doi: 10.1021/cr500648z
Hao, L., Tang, S., Yan, J., Cheng, L., Guan, S., Zhao, Q., et al. (2019). Solar-responsive photocatalytic activity of amorphous TiO2 nanotube-array films. Mater. Sci. Semiconduct. Process. 89, 161–169. doi: 10.1016/j.mssp.2018.09.014
Hong, Y., Zhang, J., Huang, F., Zhang, J., Wang, X., Wu, Z., et al. (2015). Enhanced visible light photocatalytic hydrogen production activity of CuS/ZnS nanoflower spheres. J. Mater. Chem. A 3, 13913–13919. doi: 10.1039/C5TA02500A
Hu, B., Cai, F., Chen, T., Fan, M., Song, C., Yan, X., et al. (2015). Hydrothermal synthesis g-C3N4/Nano-InVO4 nanocomposites and enhanced photocatalytic activity for hydrogen production under visible light irradiation. ACS Appl. Mater. Interfaces 7, 18247–18256. doi: 10.1021/acsami.5b05715
Ingram, D. B., Christopher, P., Jonathan Bauer, L., and Linic, S. (2011). Predictive model for the design of plasmonic metal/semiconductor composite photocatalysts. ACS Catal. 1, 1441–1447. doi: 10.1021/cs200320h
Li, M., Zhang, L., Fan, X., Zhou, Y., Wu, M., and Shi, J. (2015). Highly selective CO2 photoreduction to CO over g-C3N4/Bi2WO6 composites under visible light. J. Mater. Chem. A 3, 5189–5196. doi: 10.1039/C4TA06295G
Liu, E., Kang, L., Wu, F., Sun, T., Hu, X., Yang, Y., et al. (2013). Photocatalytic reduction of CO2 into methanol over Ag/TiO2 nanocomposites enhanced by surface plasmon resonance. Plasmonics 9, 61–70. doi: 10.1007/s11468-013-9598-7
Liu, J., Zhang, T., Wang, Z., Dawson, G., and Chen, W. (2011). Simple pyrolysis of urea into graphitic carbon nitride with recyclable adsorption and photocatalytic activity. J. Mater. Chem. 21, 14398–14401. doi: 10.1039/c1jm12620b
Martha, S., Nashim, A., and Parida, K. M. (2013). Facile synthesis of highly active g-C3N4 for efficient hydrogen production under visible light. J. Mater. Chem. A 1, 7816–7824. doi: 10.1039/c3ta10851a
Niu, P., Zhang, L., Liu, G., and Cheng, H.-M. (2012). Graphene-like carbon nitride nanosheets for improved photocatalytic activities. Adv. Funct. Mater. 22, 4763–4770. doi: 10.1002/adfm.201200922
Ong, W.-J., Tan, L.-L., Chai, S.-P., Yong, S.-T., and Mohamed, A. R. (2014). Facet-dependent photocatalytic properties of TiO2-based composites for energy conversion and environmental remediation. ChemSusChem 7, 690–719. doi: 10.1002/cssc.201300924
Park, H., Kim, H., Moon, G., and Choi, W. (2016). Photoinduced charge transfer processes in solar photocatalysis based on modified TiO2. Energy Environ. Sci. 9, 411–433. doi: 10.1039/c5ee02575c
Qi, K., Cheng, B., Yu, J., and Ho, W. (2017a). Review on the improvement of the photocatalytic and antibacterial activities of ZnO. J. Alloys Compd. 727, 792–820. doi: 10.1016/j.jallcom.2017.08.142
Qi, K., Cheng, B., Yu, J., and Ho, W. (2017b). A review on TiO2-based Z-scheme photocatalysts. Chinese J. Catalysis 38, 1936–1955. doi: 10.1016/S1872-2067(17)62962-0
Qi, K., Liu, S., Chen, Y., Xia, B., and Li, G. D. (2018a). A simple post-treatment with urea solution to enhance the photoelectric conversion efficiency for TiO2 dye-sensitized solar cells. Solar Energy Mater. Solar Cells 183, 193–199. doi: 10.1016/j.solmat.2018.03.038
Qi, K., Liu, S., and Qiu, M. (2018b). Photocatalytic performance of TiO2 nanocrystals with/without oxygen defects. Chinese J. Catalysis 39, 867–875. doi: 10.1016/S1872-2067(17)62999-1
Qi, K., Xie, Y., Wang, R., Liu, S., and Zhao, Z. (2019). Electroless plating Ni-P cocatalyst decorated g-C3N4 with enhanced photocatalytic water splitting for H2 generation. Appl. Surf. Sci. 466, 847–853. doi: 10.1016/j.apsusc.2018.10.037
Shi, T., Duan, Y., Lv, K., Hu, Z., Li, Q., Li, M., et al. (2018). Photocatalytic oxidation of acetone over high thermally stable TiO2 nanosheets with exposed (001) facets. Front. Chem. 6:175. doi: 10.3389/fchem.2018.00175
Sun, J. X., Yuan, Y. P., Qiu, L. G., Jiang, X., Xie, A. J., Shen, Y. H., et al. (2012). Fabrication of composite photocatalyst g-C3N4-ZnO and enhancement of photocatalytic activity under visible light. Dalton Transac. 41, 6756–6763. doi: 10.1039/c2dt12474b
Tao, J., Yang, M., Chai, J. W., Pan, J. S., Feng, Y. P., and Wang, S. J. (2014). Atomic N modified rutile TiO2(110) surface layer with significant visible light photoactivity. J. Phys. Chem. C 118, 994–1000. doi: 10.1021/jp408798f
Tian, K., Liu, W. J., and Jiang, H. (2015). Comparative investigation on photoreactivity and mechanism of biogenic and chemosythetic Ag/C3N4 composites under visible light irradiation. ACS Sustain. Chem. Eng. 3, 269–276. doi: 10.1021/sc500646a
Tong, T., Zhu, B., Jiang, C., Cheng, B., and Yu, J. (2018). Mechanistic insight into the enhanced photocatalytic activity of single-atom Pt, Pd or Au-embedded g-C3N4. Appl. Surf. Sci. 433, 1175–1183. doi: 10.1016/j.apsusc.2017.10.120
Wang, B., Xia, J., Mei, L., Wang, L., and Zhang, Q. (2017). Highly efficient and rapid lead(II) scavenging by the natural artemia cyst shell with unique three-dimensional porous structure and strong sorption affinity. ACS Sustain. Chem. Eng. 6, 1343–1351. doi: 10.1021/acssuschemeng.7b03667
Wang, L., Fu, H., Liu, H., Yu, K., Wang, Y., and Ma, J. (2018a). In-situ packaging ultra-uniform 3D hematite nanotubes by polyaniline and their improved gas sensing properties. Mater. Res. Bull. 107, 46–53. doi: 10.1016/j.materresbull.2018.06.034
Wang, L., Wang, S., Fu, H., Wang, Y., and Yu, K. (2018b). Synthesis of Au nanoparticles functionalized 1D α-MoO3 nanobelts and their gas sensing propertie. NANO Brief Rep. Rev. 13:1850115. doi: 10.1142/S1793292018501151
Wei, Y., Jiao, J., Zhao, Z., Liu, J., Li, J., Jiang, G., et al. (2015). Fabrication of inverse opal TiO2-supported Au@CdS core–shell nanoparticles for efficient photocatalytic CO2 conversion. Appli. Catalysis B Environ. 179, 422–432. doi: 10.1016/j.apcatb.2015.05.041
Wei, Z., Zhang, Y., Wang, S., Wang, C., and Ma, J. (2018). Fe-doped phosphorene for the nitrogen reduction reaction. J. Mater. Chem. A 6, 13790–13796. doi: 10.1039/C8TA03989E
Wen, J., Li, X., Liu, W., Fang, Y., Xie, J., and Xu, Y. (2015). Photocatalysis fundamentals and surface modification of TiO2 nanomaterials. Chinese J. Catalysis 36, 2049–2070. doi: 10.1016/S1872-2067(15)60999-8
Wen, J., Xie, J., Chen, X., and Li, X. (2017). A review on g-C3N4-based photocatalysts. Appl. Surf. Sci. 391, 72–123. doi: 10.1016/j.apsusc.2016.07.030
Wu, M., Yan, J.-M., Zhang, X.-W., Zhao, M., and Jiang, Q. (2015). Ag2O modified g-C3N4 for highly efficient photocatalytic hydrogen generation under visible light irradiation. J. Mater. Chem. A 3, 15710–15714. doi: 10.1039/C5TA03358F
Xia, Y., Li, Q., Lv, K., and Li, M. (2017). Heterojunction construction between TiO2 hollowsphere and ZnIn2S4 flower for photocatalysis application. Appl. Surf. Sci. 398, 81–88. doi: 10.1016/j.apsusc.2016.12.006
Xiang, Q., Yu, J., and Jaroniec, M. (2011). Preparation and enhanced visible-light photocatalytic H2-production activity of graphene/C3N4 composites. J. Phys. Chem. C 115, 7355–7363. doi: 10.1021/jp200953k
Xu, Q., Zhu, B., Jiang, C., Cheng, B., and Yu, J. (2018). Constructing 2D/2D Fe2O3/g-C3N4 direct Z-scheme photocatalysts with enhanced H2 generation performance. Solar RRL 2:1800006. doi: 10.1002/solr.201800006
Yan, Z., Gong, S., An, L., Yue, L., and Xu, Z. (2018a). Enhanced catalytic activity of graphene oxide/CeO2 supported Pt toward HCHO decomposition at room temperature. React. Kinet. Mech. Catalysis 124, 293–304. doi: 10.1007/s11144-018-1348-6
Yan, Z., Yang, Z., Xu, Z., An, L., Xie, F., and Liu, J. (2018b). Enhanced room-temperature catalytic decomposition of formaldehyde on magnesium-aluminum hydrotalcite/boehmite supported platinum nanoparticles catalyst. J. Colloid Interface Sci. 524, 306–312. doi: 10.1016/j.jcis.2018.04.018
Yang, S., Gong, Y., Zhang, J., Zhan, L., Ma, L., Fang, Z., et al. (2013a). Exfoliated graphitic carbon nitride nanosheets as efficient catalysts for hydrogen evolution under visible light. Adv. Mater. 25, 2452–2456. doi: 10.1002/adma.201204453
Yang, S., Wang, H., Yu, H., Zhang, S., Fang, Y., Zhang, S., et al. (2016). A facile fabrication of hierarchical Ag nanoparticles-decorated N-TiO2 with enhanced photocatalytic hydrogen production under solar light. Int. J. Hydrogen Energy 41, 3446–3455. doi: 10.1016/j.ijhydene.2015.12.190
Yang, Y., Guo, Y., Liu, F., Yuan, X., Guo, Y., Zhang, S., et al. (2013b). Preparation and enhanced visible-light photocatalytic activity of silver deposited graphitic carbon nitride plasmonic photocatalyst. Appli. Catalysis B Environ. 142–143, 828–837. doi: 10.1016/j.apcatb.2013.06.026
Zhang, K. M., and Wen, Z. G. (2008). Review and challenges of policies of environmental protection and sustainable development in China. J. Environ. Manage. 88, 1249–1261. doi: 10.1016/j.jenvman.2007.06.019
Zhong, Y., Liu, Y., Wu, S., Zhu, Y., Chen, H., Yu, X., et al. (2018). Facile fabrication of BiOI/BiOCl immobilized films with improved visible light photocatalytic performance. Front. Chem. 6:58. doi: 10.3389/fchem.2018.00058
Zhou, X., Luo, Z., Tao, P., Jin, B., Wu, Z., and Huang, Y. (2014). Facile preparation and enhanced photocatalytic H2-production activity of Cu(OH)2 nanospheres modified porous g-C3N4. Mater. Chem. Phys. 143, 1462–1468. doi: 10.1016/j.matchemphys.2013.11.066
Keywords: Ag nanoparticles, Ag/g-C3N4, photocatalytic activity, organic pollutant, electron-hole separation, modification
Citation: Qi K, Li Y, Xie Y, Liu S, Zheng K, Chen Z and Wang R (2019) Ag Loading Enhanced Photocatalytic Activity of g-C3N4 Porous Nanosheets for Decomposition of Organic Pollutants. Front. Chem. 7:91. doi: 10.3389/fchem.2019.00091
Received: 31 August 2018; Accepted: 04 February 2019;
Published: 02 April 2019.
Edited by:
Bing Li, Institute of Materials Research and Engineering (A*STAR), SingaporeReviewed by:
Priyabrat Mohapatra, C. V. Raman College of Engineering, IndiaWenbo Wang, Lanzhou Institute of Chemical Physics (CAS), China
Copyright © 2019 Qi, Li, Xie, Liu, Zheng, Chen and Wang. This is an open-access article distributed under the terms of the Creative Commons Attribution License (CC BY). The use, distribution or reproduction in other forums is permitted, provided the original author(s) and the copyright owner(s) are credited and that the original publication in this journal is cited, in accordance with accepted academic practice. No use, distribution or reproduction is permitted which does not comply with these terms.
*Correspondence: Shu-yuan Liu, liushuyuan@symc.edu.cn
Zhe Chen, chenzhecz999@163.com
Ruidan Wang, wangruidan1980@163.com
†These authors have contributed equally to this work