Using Functional Trait Diversity Patterns to Disentangle the Processes Influencing the Recovery of Subalpine Grasslands Following Abandonment of Agricultural Use
- 1Institute of Tropical Agriculture and Forestry, Hainan University, Haikou, China
- 2Department of Biological Sciences, Indian Institute of Science Education and Research, Kolkata, India
- 3State Key Laboratory of Grassland Agro-ecosystems, School of Life Sciences, Lanzhou University, Lanzhou, China
Grasslands in the Qinghai-Tibetan Plateau, as in much of the world, are increasingly degrading due to exploitation for agriculture and grazing. Restoring such grasslands or facilitating recolonization to create native late-successional communities requires an understanding of how communities within grasslands are structured within a secondary succession series. Here we studied community assembly using species abundance, soil nutrient levels, three functional traits (photosynthesis rate, seed mass, and seed germination rate) in two comparable chronosequences of sub-alpine grasslands in the Qinghai-Tibetan Plateau. These grasslands range from “natural grassland” (without human management), to those that have been excluded from agricultural exploitation for 4–13 years. We were interested in shifts in functional trait diversity (FD; the value and range of functional traits in a community) and trait dispersion represented by the differences between observed FD and the expectation under neutral assembly. We found that a number of abiotic and/or biotic filters significantly influenced the success of species in different successional states, leading to systematic shifts in plant life history strategies, from traits indicating high relative growth rates to traits indicating high competitiveness. We also found comparable community assembly processes between 13-year and natural grasslands, where hierarchical competition led to the exclusion of forb species by graminoid species and facilitation induced by legume species. Our results have three implications for degraded subalpine grasslands caused by agriculture exploitation in Qinghai Tibetan plateau. First, in the course of the abandonment of agricultural use and the return from degraded to natural grasslands, deterministic processes induced a shift in plant strategies from rapid relative growth rate under low N competition to slow relative growth rate under high N competition. Second, active seeding of graminoid and legume species may reduce the hierarchical competition, which in turn speedup recovery from agriculture exploitation. Finally, due to the close connection of functional attributes (e.g., growth, colonization, recruitment, and resource competition) and community structure, monitoring FD and trait dispersion patterns may be a good way to evaluate the restoration efforts in degraded grasslands caused by agriculture exploitation in Qinghai Tibetan plateau.
Introduction
Historic human impacts (i.e., agricultural exploitation) have led to destruction and degradation of grasslands threatening global biodiversity (Chapin et al., 2000). Nevertheless, human land use can also increase α-biodiversity at landscape level in some areas by creating immediate gaps for additional competitively weak plant species or by accelerating species differentiation in some taxonomic groups in the long term (Ellenberg, 1996). In recent decades, rapid degradation has been observed in the Qinghai Tibetan plateau (Millennium Ecosystem Assessment, 2005; Lue et al., 2011). It has been shown that rates of degradation in these grasslands have increased by 13–29% over the last 30 years (Lue et al., 2011). If these high rates of degradation remain unchecked, recovery of these degraded grasslands could be slowed or they could be lost to other land use (Zhang et al., 2013).
The degradation of grasslands in Qinghai Tibetan Plateau may also constitute an increasing source of carbon emissions to the atmosphere. Conversely, restoring the severely degraded lands to perennial vegetation is a valuable approach to sequestering carbon in degraded systems (Wang et al., 2005). Therefore, understanding the processes of grassland plant community recovery and restoration has been identified as a major priority for the Qinghai Tibetan Plateau (Li et al., 2013). More generally, extensive degradation of grasslands has become a global problem (Millennium Ecosystem Assessment, 2005). Therefore, such research is pertinent for other regions where restoration is a key component of planning and development.
However, without a clear understanding of the mechanisms driving secondary grassland succession, predicting successional trajectories and understanding how communities recover from degradation will remain a difficult task (Prach and Walker, 2011). Such understanding of successional processes has increased during the past decade by the use of trait-based approaches to community assembly (Mason et al., 2012; Zhang et al., 2015). The deterministic view is that for community assembly during secondary succession, a series of successive abiotic and/or biotic filters select species with specific life histories (e.g., resource competitive ability and stress tolerance) (Bazzaz, 1975; Levine and Murrell, 2003), which should be associated with predictable change in functional trait diversity (FD) during secondary succession (Petchey and Gaston, 2006). Here FD represents the “intra-community values and ranges” of functional traits, which are components of an organism's phenotype that in turn influence ecosystem level processes (Lohbeck et al., 2012; Bu et al., 2014).
Secondary succession in Tibetan highland grassland begins with colonization, following a stand-replacing disturbance, and the early colonists (e.g., forb species) are expected to possess high colonizing abilities (i.e., high dispersal and fast-growing abilities) (Marcante et al., 2009; Zaplata et al., 2011, 2013). Thus, initial colonists are expected to show high relative growth rates given the typical high resource availability (e.g., light and soil nutrients) at early states (Zhang et al., 2018). This similarity in life histories and resource requirements of the early colonists should result in trait convergence (observed FD is less than expected if trait values were randomly distributed), with abiotic filtering playing a strong role during early succession (Grime, 2006). However, resource limitation is expected to develop over time, and trait convergence should weaken over time in secondary succession. Trait divergence should develop if competitive interactions among initial colonists select dissimilar species in late-successional communities (Grime, 2006; Mason et al., 2012). When slower-dispersing but more strongly competitive species (e.g., graminoid species) arrive in late succession, initial colonists will be gradually excluded by the strong competitors (Hutchinson, 1951; Pulsford et al., 2016) and the resultant hierarchical competition (species with highly competitive traits to exclude others with less-competitive traits) should cause trait convergence (Mayfield and Levine, 2010; Kunstler et al., 2012) (Figure 1).
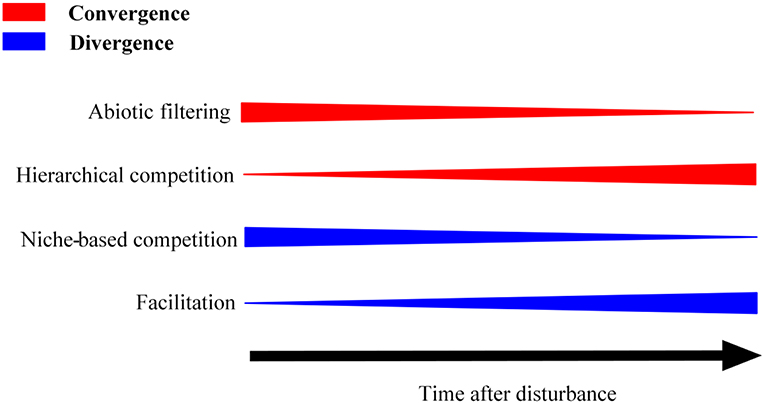
Figure 1. Hypotheses concerning patterns in the relationship between trait dispersion (convergence and divergence) and community assembly processes during secondary succession.
Compared to species that face resource limitation or environmental harshness, species in high stress environments (i.e., intensive and prolonged UV radiation, extremes of temperature, and limited precipitation) such as the Qinghai-Tibetan plateau are likely to experience interspecific facilitation (Chu et al., 2008). Due to the greater competition between closely related species, interspecific facilitation may involve distantly related species during secondary succession (Verdú et al., 2009) and cause trait divergence. However facilitation may take place even between closely related species (e.g., congeneric species) if these species show divergent traits thereby reducing niche overlap (Beltrán et al., 2012). In addition the abiotic environment can also influence the link between interspecific facilitation and trait dispersion in the resultant community (Lin et al., 2012; Gallien et al., 2018). Chu et al. (2008) report that interspecific facilitation was trigged by cold stress in Tibetan highland grassland and therefore should result in trait divergence in this plant community. Therefore, if interspecific facilitation is the dominant community assembly mechanism during secondary succession, trait divergence should increase with successional age (Figure 1). These distinct patterns of trait dispersion could be expected when different assembly mechanisms dominate secondary succession. Consequently, quantifying the patterns of FD and trait dispersions of different successional grasslands could provide insight on the mechanisms of secondary grassland succession in Qinghai Tibetan plateau.
Here we use a multi-level sampling approach to study community assembly during secondary grassland succession after release from agriculture (previously plowed and agriculturally used) by testing FD patterns in a number of subalpine ecosystems. In our previous work in grasslands undergoing succession we found that the rate of sub-alpine grassland restoration following protection from farming is rapid, occurring within a short period of about 10 years (species composition was similar between 10-year grassland and natural grasslands (without human management) (Zhang et al., 2013). By measuring functional traits that have direct influence on survival, growth, and reproduction, we can test mechanisms of community assembly in action during succession (McGill et al., 2006; Cadotte et al., 2011). We therefore compiled a functional trait dataset including one physiological trait (photosynthesis rate), and two reproductive traits (seed mass and seed germination rate in the lab), and the choice of these functional traits was based on the unique environmental characteristics in the Qinghai-Tibetan Plateau — intensive and prolonged UV radiation, and the vast open grassland landscape (Klein et al., 2004). The specific questions that we test are (1), do grasslands show directional shifts in plant life history strategies manifest in FD for three key traits after release from agriculture, and (2) do the underlying mechanisms that determine trait dispersion patterns within grasslands change in importance as these areas recover from agriculture? The answers to these questions may indicate the possible mechanisms that select plant life history strategies and influence community assembly following protection from agricultural exploitation in the Qinghai-Tibetan plateau. We also propose to use the insight gained to propose useful restoration strategies for the recovery of degraded grasslands.
Materials and Methods
Study Site
Sampling was carried out in the eastern part of the Qinghai-Tibetan Plateau, Hezuo, China (34°55′N, 102°53′E) at a mean elevation of ~3,000 m above sea level. This region is characterized by a cold dry climate, with a mean annual temperature of 2.4°C, and a mean annual precipitation of 530 mm distributed mainly during July-August. Local vegetation including in natural sites is dominated by graminoid species, such as Elymus nutans (Griseb), Kobresia pygmaea (C. B. Clarke), and Thermopsis lanceolata (R. Br) (Zhang et al., 2012). Historic human impacts mainly by agricultural exploitation have caused serious land degradation (Wang et al., 2005). In response, local governments have constructed fences to restrict agriculture to designated areas. These measures allowed succession and were thus responsible for the emergence of chronosequences, such as the ones we use in our study. All our sampled sites, except the control grasslands (without human managements for at least 40 years), had been used for agriculture to grow highland barley in the recent past, with cessation of cultivation within the last 4–13 years. Recovering grasslands were classified under one of four “successional ages” (4-, 6-, 10-, and 13-year), based on the number of years since cultivation had ceased.
In earlier work we reported that recovery of grasslands may occur within 10 years (Zhang et al., 2013) and that species composition was similar between 1- and 3-year grasslands (as of 2009). Counting 3 additional years of time elapsed since 2009 to our 2013 sampling; we define 4- and 6-year grasslands as early successional grasslands and 10- and 13-year grasslands as late-successional grasslands. The time since the end of agricultural use has been determined by interviews with local farmers.
Field Sampling
Sampling was conducted in August (the peak of the growing season) of 2013 in the study grasslands. Within a large area near Hezuo city, we identified two spatially distinct study areas for which farming histories could be reliably obtained by interviewing local farmers. These two areas (named chronosequence 1 and chronosequence 2) were 10 km apart and possessed comparable topographic characteristics (e.g., orientation and slope) and sites representing each of the five successional ages of interest (Figure 2). We therefore sampled two independent replicates for each of the five successional ages, yielding a total of 10 sites (successional grasslands).
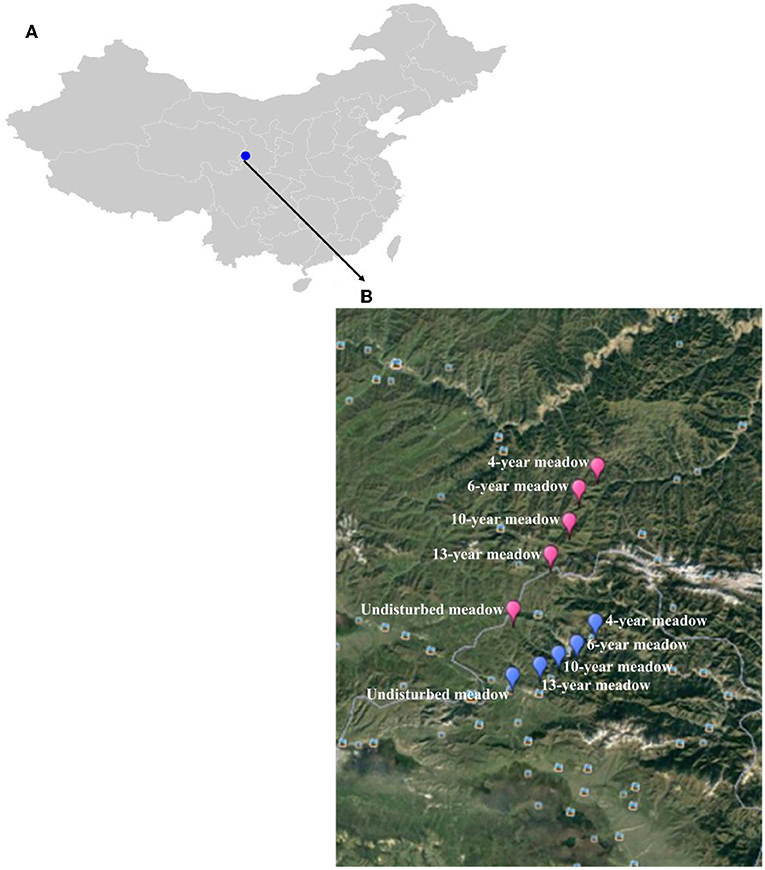
Figure 2. Location map of study sites in the eastern part of the Qinghai Tibetan plateau, Hezuo, China. (A) Hezuo city (B) locations of ten sites representing each of the five successional ages (4-, 6-, 10-, 13-year and natural grassland).
At each site we randomly selected an identified area of 120 × 120 m. Subsequently, we arranged 30 quadrats (0.5 × 0.5 m) regularly in six parallel transects, maintaining with 20 m intervals separation between two adjacent sampling quadrats. In each quadrat, we clipped the aboveground parts of each vascular plant species and transported to the laboratory to determine aboveground biomass. This was determined as dry weight by oven-drying samples at 105°C for 2 days. Species abundance for species at each successional age was quantified as the total value of aboveground biomass for each individual species found within all 30 quadrats of each site.
Soil Variables Measurement
We collected several microsamples in each of the 30 quadrats per site and averaged across all sites of the same successional age to cumulate sampled soil up to 50 g of topsoil (0–20-cm depth). We used 20 g of sampled topsoil to measure soil water content gravimetrically. The remaining 30 g of sampled topsoil was used for measurement of the important macro- and micro-nutrients required for plant growth. The elements quantified were, total nitrogen, phosphorus, potassium, calcium, sulfur, magnesium, sodium, iron, copper, boron, zinc, manganese, and molybdenum; all of which were quantified using X-ray diffraction on an auto analyzer (D8 DISCOVER X-ray diffraction, Bruker, CO, Germany). Soil carbon was also measured similarly. Soil pH was measured using a pH meter (UB-7, UltraBASIC, Denver, CO, USA) after suspending the soil in KCl.
Collection of Functional Traits
We quantified plant relative growth rate via the photosynthesis rate. We further quantified two reproductive traits (seed mass and seed germination rate), which are known to influence multiple life historical attributes of a plant, including dispersal, recruitment potential, stress tolerance, and resource competition. Importantly, for species that occurred in multiple grasslands of different successional ages, we measured the functional traits in all grasslands to ensure that intraspecific variation was appropriately incorporated into our analyses. The specific details for measuring these three traits are given in the Supplementary Material.
Statistical Methods
Variation in Functional Trait Diversity for Each of the Three Traits During Succession
We first tested the correlation between functional trait diversity (FD) and each of the three traits (photosynthesis rate, seed mass, and seed germination rate) as a function of successional age within the two successional chronosequences. Successional age was rank-transformed to include the natural grassland as a control. Setting the control grassland to a high succession age value (>40 years) yielded qualitatively similar and statistically significant results (P < 0.05). However, the amount of variation explained was much lower and resulted in an extremely low influence of control grassland data points (Table S1). Estimating functional diversity using multiple traits has been criticized (Chalmandrier et al., 2017), since traits may represent different niche axes and the aggregated information may obscure certain assembly rules (Spasojevic and Suding, 2012). Here we chose to assess FD through mean pairwise distance (MPD) as our FD index, following de Bello (2012):
where, dij is the dissimilarity between species i and j. Pi and Pj is the relative abundance of species i (i = 1, 2, ….,s) and species j (j = 1,2, ….,s) respectively. The values of dij ranges from 0 to 1 and is based on using a set of specific functional trait to calculate Gower's similarity index (Ricotta and Moretti, 2011). Evidently, dij = 0, indicates that trait diversity of species i is the same as that of species j, and dij = 1 indicates that trait diversity of species i is totally different from species j. We chose MPD because the selected traits (photosynthesis rate, seed mass, and seed germination rate) are recognized to represent orthogonal axes of plant strategies and MPD allows determination of FD for each trait (de Bello et al., 2012). Furthermore, this index has been shown to be independent of species numbers (Pavoine and Bonsall, 2011). Using this index to measure FD therefore minimizes the influence of differences in species numbers.
Trait Dispersion Patterns and Their Determinants
We first compare the observed FD for each of the three traits within communities to those simulated by random sampling within a subplot, to test whether the observed trait dispersion was the result of random distribution, trait convergence, or trait divergence. The method proposed by Legendre et al. (2009) employs distance-based Moran's eigenvector maps (MEM) and redundancy analysis-based variance partitioning (RDABVP) to partition FD into variations related to abiotic filtering (due to measured environmental variables) and dispersal limitation (due to pure spatial variables) (Myers et al., 2013). We, therefore, used MEM and RDABVP to quantify the relative contribution of abiotic filtering represented by spatial soil and topographic heterogeneity and dispersal limitation manifest in MEM to FD. On the other hand, the combination of MEM and RDABVP allowed for disentangling the coupling influences of abiotic filtering and weaker competitor exclusion on trait convergence (Zhang et al., 2018). The specific details for performing these two FD-based tests are as follows.
Comparing the Observed Functional Trait Diversity to Those From Null Communities
To test whether any observed trait dispersion pattern shows convergence or divergence or does not significantly differ from a random distribution at each successional age, we simulated null communities in which species and trait values were randomly distributed. Randomization procedures were applied to calculate “null” distributions for both species composition (i.e., on the species × quadrat matrix) and FD of the all species (de Bello, 2012). Reshuffling the species × quadrat matrices at each successional grassland was performed simultaneously with three constraints: (i) the same number of species (species richness) per plot in the simulated and observed data; (ii) the same number of total species occurrences per region (i.e., number of plots where the species occur in a successional grassland); and (iii) a constant total abundance of species in a region (i.e., the sum of the number of quadrats occupied in all plots). We implemented the randomization procedures using the function “randomizeMatrix” in the “picante” package (Kembel, 2010). We then compared the observed FD to the simulated FD simulated in 1,000 randomly assembled communities. Specifically, we computed the standard effect size index (SES) following Gotelli and McCabe (2002):
where FDobs and FDrandom represent observed FD and mean simulated null community FD values, respectively. FDsd represents the standard deviation of simulated FD values. Wilcoxon signed-rank tests were performed to test whether the SES significantly differed from zero, and when it did differ from zero, whether SES was significantly more than or less than zero. Non-significant differences from zero, significantly greater than zero, and significantly less than zero, are indicative respectively of a random distribution, prevalence of trait divergence, and prevalence of trait convergence (de Bello, 2012).
Partitioning the Spatial Patterns of Functional Trait Diversity
We used Moran's eigenvector maps (MEM) to quantify spatial autocorrelations in FD represented by MPD for each of the successional grasslands. MEM was based on the principal coordinates of neighbor matrix (PCNM) axes (Legendre and Legendre, 2012) and could be used to describe the spatial autocorrelations in FD (Zhang et al., 2015). We firstly use the function of “cmdscale” in “SpacemakerR” package in R (Stephane, 2013) to calculate MEM. We then used the R function “poly” to quantify the polynomial terms for each variable including both linear and non-linear relationships between abiotic variables and FD. While performing redundancy analysis (RDA) based variance partitioning, we first used the method developed by Blanchet et al. (2008) to forward-select significant abiotic variables among the measured soil variables (soil total N, C, P, K, Ca, S, Mg, Na, Fe, Cu, B, Zn, Mn, and Mu) and pure spatial variables represented by MEM, associated with FD across spatial scales. For the significant abiotic variables, we used box plots to examine trends in means and variances with an increase in spatial scales. Then, we used variance partitioning to allocate FD variation to four complementary components: (a) “purely abiotic variables” (explained by abiotic factors only), (b) “spatially structured abiotic variables” (explained by both abiotic variables and spatial autocorrelation in FD), (c) “purely spatial variables” (explained by spatial autocorrelation in FD only), and (d) “undetermined variables” (Legendre et al., 2009; Siefert, 2012). At each spatial scale, variance partitioning was done using the function of “varpart” in “vegan” package in R (Oksanen et al., 2016). Specifically, variations in observed FD explained by both purely environmental variables and spatially structured abiotic variables represent the relative contributions of environmental variables to observed FD.
Results
Species richness increased from 61 to 82 species during secondary succession, with 50 species sharing among all five successional grasslands (Table S2). Moreover, species composition was almost identical between 4- and 6-year grasslands, with 60 species sharing between these two grasslands (Table S2). Similar patterns were found in late successional grasslands, with 70 species shared among 10-, 13-year, and natural grasslands (Table S2).
We first tested for directional shifts in functional trait diversity (FD), as measured by mean pairwise distance (MPD), for photosynthesis rate among these secondary successional grasslands. We found that FD for photosynthesis rate, decreased significantly with successional age at both sites (P < 0.01, Figures 3A,B). For seed mass and seed germination rate the FD increased significantly with successional age at both sites (P < 0.01, Figures 3C–F).
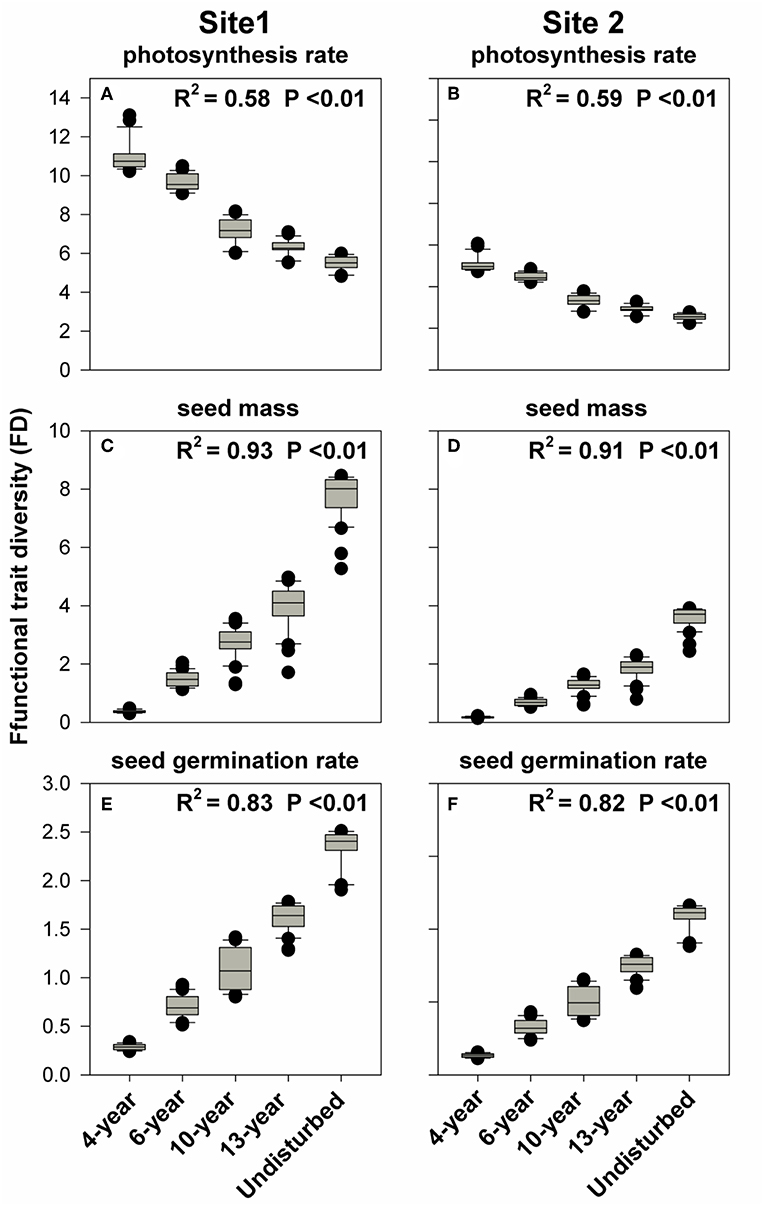
Figure 3. The variations in FD for photosynthesis rates, seed mass, and seed germination rates for the successional meadows. (A,C,E) Represent FD for photosynthesis rate, seed mass, and seed germination rate in chronosequence 1, respectively. Likewise, (B,D,F) indicate FD for photosynthesis rate, seed mass, and seed germination rate in chronosequence 2 individually.
We then investigated how communities within these successional grasslands are assembled, by testing trait dispersion patterns. We found significant convergence for all three traits, i.e., the SES values were significantly less than zero, for the 4-year and late successional (10-, 13-year, and natural grassland) site replicates (Figure 4). Significant trait divergence, i.e., the SESs significantly greater than zero, was observed only for the 6-year successional replicate sites for all three traits (Figure 4).
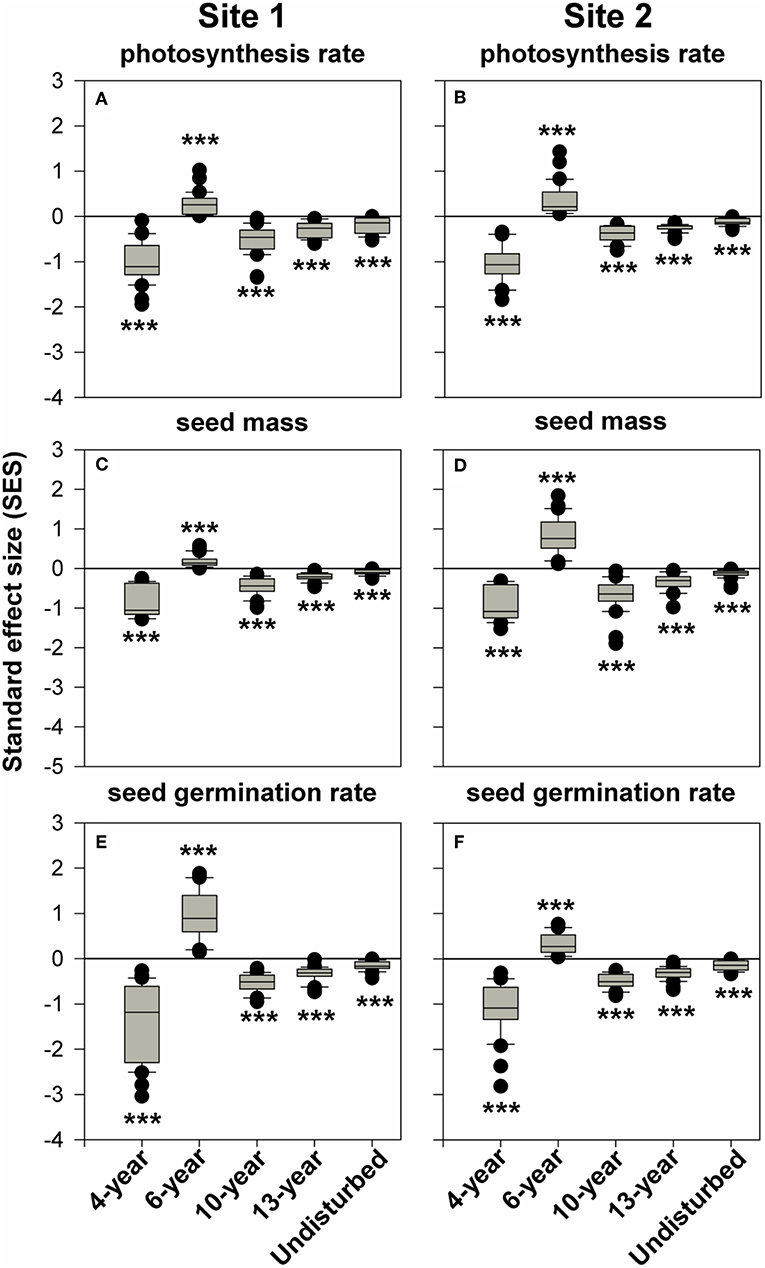
Figure 4. The distributions of standard effect size (SES) in successional meadows. ***P < 0.001 for the Wilcoxon signed-rank test, for null hypothesis that SES is equal to zero. SES values significantly greater than, smaller than, or not different from zero, respectively, indicate significant trait divergence, trait convergence, or random distribution. The bottom and top of the box represent the 1st quartile and 3rd quartile of the data, respectively. The vertical lines extending from the box represents the data inside a range of 1.5 times the interquartile range from the box. Outliers are denoted by filled points. (A,C,E) Represent SES for photosynthesis rate, seed mass, and seed germination rate in chronosequence 1, respectively. Likewise, (B,D,F) indicate SES for photosynthesis rate, seed mass, and seed germination rate in chronosequence 2 individually.
We also used variance partitioning analysis to test the relative contributions of 11 soil variables to observed FDs for these three traits. We found that, in 4-year successional grasslands, soil variables (non-spatial and spatially-structured soil variables) explained large proportions (from 53 to 64%) of the variations in FDs for all three traits examined (Figure 5), whereas pure spatial variables explained only small proportions (from 4 to 16%) of the variation. However, pure spatial variables were more important for successional grasslands older than 6 years, explaining 33–53% of the variations in FD. Soil variables had less explanatory power (from 5 to 26% of the total variation) in comparison (Figure 5).
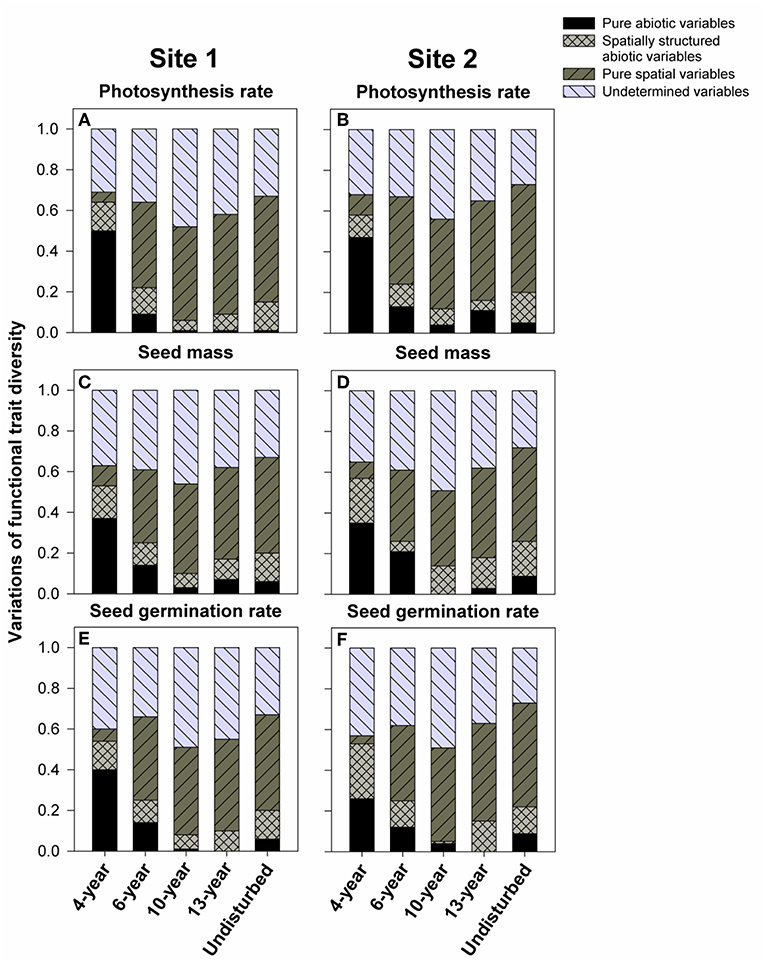
Figure 5. Variance partitioning analyses of the distributions of functional trait diversity with successional age. Variations explained by purely abiotic, spatially structured abiotic, purely spatial, or undetermined variables are shown if statistically significant (p < 0.05). Each analysis was performed on all quadrants within the successional grasslands. (A,C,E) Represent variations of FD for photosynthesis rate, seed mass, and seed germination rate in chronosequence 1, respectively. Likewise, (B,D,F) indicate variations of FD for photosynthesis rate, seed mass, and seed germination rate in chronosequence 2 individually.
Moreover, our forward selection regression analyses showed that soil pH, SWC, C, P, K, Ca, S, Mg, Na, Fe, Zn, and Mg were significant predictors of FDs for the three traits only in some cases, only soil nitrogen has always been significant in all cases (Tables S3–S5). We therefore used box plots to examine trends in means and variances in soil N with increase in successional age. We found soil N values across replicate sites initially decreased with successional age up to 10-year old grasslands (Figures 6A,B), and thereafter increased from 13-year to natural grasslands (Figures 6A,B). However, soil N values for 13-year and natural grasslands never exceeded the initial (4- and 6-year) values (Figures 6A,B).
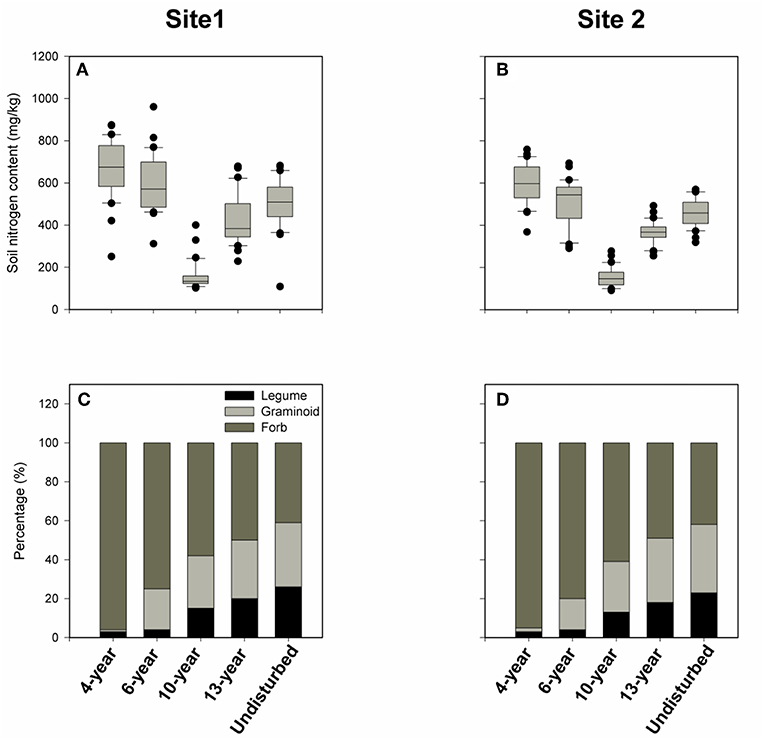
Figure 6. Box plots of variation in soil total nitrogen and the respective percentages of the three main function groups (forb, graminoid, and legume species) among the 30 quadrats in each grassland of each successional age. (A,C) Represent soil nitrogen content and the respective percentages of the three main plant functional groups (legume, graminoid, and forb) in chronosequence 1, respectively. Likewise, (B,D) indicate soil nitrogen content and the individual percentage of the three main plant functional groups (legume, graminoid, and forb) in chronosequence 2 individually.
Finally, we tested whether the observed variation in nitrogen availability, FD, and trait dispersion were related to relative abundance of the three main functional groups (non-leguminous forb species, graminoid, and legume species) across successional age groups. We found that the aboveground biomass percentage of non-leguminous forb species decreased from 96 to 41% during secondary succession. The aboveground biomass proportions of both graminoid species (from 2 to 35%) and legume species (from 2 to 24%) increased with successional ages in both sites (Figures 6C,D). Since the highest aboveground biomass proportions for graminoid and legume species were only 35 and 24% respectively, non-leguminous forb species still made up the highest percentage of aboveground biomass in all successional grassland communities in both sites (Figures 6C,D).
Discussion
Community assembly during secondary succession often reflects an a shift in plant strategies, from high relative growth rate under low resource competition to low relative growth rate under high resource competition, with concomitant changes in FD (Granath et al., 2010; Zhu et al., 2013). Since photosynthesis rates, seed mass and germination can be associated with a resource acquisitive-conservation trade-off during succession (Coomes and Grubb, 2003; Lohbeck et al., 2013), and degraded grasslands can return to natural conditions after abandonment of use, a significant change in the FD for these traits is observed. Consequently, the directional changes in FD patterns for three important traits over succession indicate that deterministic processes dominate the recovery after release from agriculture (Lohbeck et al., 2014).
Our trait dispersion patterns (for photosynthesis rate, seed mass and seed germination rate) reveal the deterministic processes (abiotic filtering and biotic interactions) that influence community assembly during secondary succession. As shown in Figure 1, the observed trait convergence within early and later succession age grasslands (4-, 10-, and 13- year, and natural grasslands) could have been due to both abiotic filtering and hierarchical competition (Kraft and Ackerly, 2010; Mayfield and Levine, 2010; de Bello et al., 2012). However, the variance partitioning results for the present study showed soil variables to explain large proportions (53–64%) of variations in the FDs of all the three traits within the 4-year successional grassland. This supports a strong role for abiotic filtering on FD for these three traits. In comparison, abiotic filtering only explained small proportions (from 5 to 26%) of the variations in FDs within grasslands of 10-year and later successional ages, including natural grasslands, which argues for a greater influence of hierarchical competition in late-succession. For 6-year old grasslands, the SES analysis showed significant divergence for all three traits, which may result from niche-based competition or facilitation as the dominant influence on community assembly. If interspecific facilitation were strongest, trait divergence would increase with increasing successional age from 6-year old to the natural grasslands. However, we observed a shift from trait divergence to convergence with increasing successional age. This pattern suggests that competitive interactions remained important in 6-year old and older grasslands, and that increasing trait convergence could be interpreted as shift in the nature of competitive interactions, from niche-based in 6-year grasslands to hierarchical in older grasslands (Hutchinson, 1951).
Soil N availability and functional group composition (non-leguminous forb, graminoid, and legume species) across successional ages could provide a possible explanation for the observed variation in FD and trait dispersion patterns (for photosynthesis rate, seed mass, and seed germination rate) during secondary succession. Soil N levels were highest in 4-year old grasslands and tests of abiotic effects on FD showed that total soil N was the only significant factor among the variables we studied. High levels of N in 4-years grasslands were expected given the recent history of cultivation. The strong abiotic effects at early successional stages is evidence that species that thrive in high resource environments dominate at early stages (Bazzaz and Pickett, 1980; Poorter et al., 2004). These species tend to have high photosynthesis rates, as we observed for the forb species-dominated 4-year old grasslands. High levels of N could eliminate competition for nitrogen and lead to low FD for seed mass and germination rates. In 6-year grasslands soil nitrogen was lower than in 4-year grasslands and the observed trait divergence could be interpreted as due to niche-based competition under low N concentration (Figure 6). Meanwhile, strong competition for N could also influence shifts to species with high seed mass and seed germination rates, leading to greater competitive ability for N but accompanied by low photosynthesis and low relative growth rates (Coomes and Grubb, 2003; Turnbull et al., 2008; Orrock and Christopher, 2010).
Soil N was lowest in the 10-year old grasslands and this can explain the functional turnover with competitively superior graminoid species replacing forb species, driven by a shift from competitive niche differentiation among forb species to hierarchical competitive exclusion of forb species by graminoid species (Grime, 2006; Mason et al., 2012). Soil N increased with successional age from 13-year to natural grasslands, but never attained the initial, early succession levels. This increase in soil N was matched by a corresponding increase in the proportions of N-fixing leguminous plants. The increase in N-fixers with succession might also have caused strong facilitation between legume and graminoid species for this subalpine grassland (Xu et al., 2010; Zhang et al., 2015), which would slow the exclusion of weak competitors (forb species), thereby weakening the effects of hierarchical competition in the 13-year and natural grasslands. This may account for the re-emergence of high proportions (from 41 to 50%) of forb species in the 13-year and natural grasslands. Indeed, there is a species turnover in non-leguminous forbs between earlier and later secondary succession (Table S2).
Altogether, it was the relatively high N availability following the cessation of agriculture that might explain the strong abiotic filtering and prevalence of high relative growth rate within 4-year old grasslands. However, N limitation can develop quickly, and competitive interactions (niche-based and hierarchical competition) between forb and graminoid species may assume importance. With the appearance of N-fixing legume species, interspecific facilitation emerged as an important contribution to assembly processes in older (10-, 13-years, and natural grassland) grasslands. However, the interaction between soil N and variations in percentages for the three main groups (forb, legume, and graminoid) is very complex. Moreover, the observed convergence and divergence in FD over secondary succession can only be treated as the inference of the influences of N-filtering and biotic interactions on community assembly during secondary succession (Zhang et al., 2015). Thus, future N-addition and functional group removal experiments are needed to test the effects of N-filtering, niche-based competition, hierarchical competition and interspecific facilitation on community assembly during secondary succession.
Our results provide insights on how to restore grasslands in the Qinghai Tibetan Plateau, with potentially wider significance for similar ecosystems. In the Qinghai-Tibetan plateau, agricultural exploitation is widespread, and these activities are degrading the biological and economic viability of these unique grasslands (Li et al., 2013). Hence, arresting further degradation and developing viable restoration methods are predominant concerns in the region. Forb, legume, and graminoid species are the main functional groups in natural grassland communities globally (Tilman et al., 2001; Stevens et al., 2004), but their proportions can also vary between and within ecosystems, biotopes and patches (Diaz et al., 2007; Biber et al., 2013; Tóth et al., 2016; Liu et al., 2017). In the Qinghai Tibetan Plateau, graminoid and legume species are rare in highly degraded grasslands, whereas graminoid and legume species are abundant in natural grassland (Wang et al., 2009). It has been shown that N is the main limiting resource that controls the functional group composition of grassland communities (Tilman, 1987; Duprè et al., 2010; Humbert et al., 2016). Hence understanding how N availability controls these three groups during secondary succession is key to develop restoration methods. For primary succession it was shown that herbaceous legumes can have their maximum in severe nutrient deficiencies, especially N (e.g., Zaplata et al., 2013). By testing the relationships between traits and environmental variables, we have shown changing N availability differently favors the three functional groups. In highly degraded grasslands, sequential seeding will help speedup recovery, as chance colonization is mostly a slower process. For example, hay seeding has been very successfully applied in grassland restoration (Donath et al., 2006). Thus, transplanting seedlings of legume species in early stages needs to be experimented for its influence on the process of grassland recovery. Because soil N reconstruction is needed to facilitate establishment of graminoid species in highly degraded grasslands, planting seeding of legume species at early stages may prove to be useful (Wang et al., 2005). As we show, legume species can also slow the competitive exclusion of forb species by graminoid species, thereby maintaining high species diversity in the natural grasslands. Hence, if recolonization rates are diminished due to isolation or other factors, active seeding of graminoid and legume species may reduce the hierarchical competition, which in turn speedup recovery from disturbance. However, further experimental studies are needed to strengthen these observations on possible trajectories for restoring degraded grasslands.
Finally, our data has notable limitations. We included only 10 study sites although from two independent successional chronosequences, which might limit the power of our analyses. Moreover, we only measured three functional traits that capture important aspects of resource competition, growth, and reproduction. Other functional attributes that reflect energy trade-offs and allocation, plant defense, and root functioning which are important and can also influence community assembly during secondary succession. These aspects of the recovery of the Qinghai-Tibetan grasslands surely merit detailed investigation.
Conclusions
Our results have three implications for degraded subalpine grasslands in Qinghai Tibetan plateau and allow a first derivation of interventional options for the restoration of degraded grasslands. First, deterministic processes induced a shift in plant strategies, from high relative growth rate under low resource competition to low relative growth rate under high resource competition dominate in the recovery of degraded grasslands to return to natural grasslands. This understanding improves our predictive abilities and suggests interventional options for the restoration of degraded grassland lands in the Qinghai-Tibetan Plateau. Second, since graminoid and legume species assume greater importance, active seeding of graminoid and legume species may speedup recovery from disturbance if dispersal limitation is severe and recolonization rates are diminished due to isolation or other factors. Finally, due to the close connection of functional attributes (e.g., growth, colonization, recruitment and resource competition) and the natural plant community, monitoring FD and trait dispersion patterns may be a good way to evaluate the restoration efforts in degraded grasslands in Qinghai Tibetan plateau. However, our data has limitations as we include only 10 study sites although from two independent successional chronosequences, which might limit the power of our analyses. Moreover, we only measured three functional traits that capture important aspects of resource competition, growth, and reproduction. Other functional attributes that reflect energy trade-offs and allocation, plant defense, and root functioning which are important and can also influence community assembly during secondary succession. This merit further investigation too.
Author Contributions
HZ and WL designed the research. HZ, RJ, KL, WQ, and WL performed the research. HZ and WL analyzed the data. HZ, RJ, and WL wrote the paper.
Funding
This work was funded by the National Natural Science Foundation of China (31770469) and the start-up fund from Hainan University [KYQD (ZR) 1876].
Conflict of Interest Statement
The authors declare that the research was conducted in the absence of any commercial or financial relationships that could be construed as a potential conflict of interest.
Acknowledgments
We would also like to thank Christine Verhille at the University of British Columbia for her assistance with English language and grammatical editing of the manuscript.
Supplementary Material
The Supplementary Material for this article can be found online at: https://www.frontiersin.org/articles/10.3389/fevo.2019.00128/full#supplementary-material
References
Bazzaz, F. (1975). Plant species diversity in old-field successional ecosystems in southern Illinois. Ecology 1, 485–488. doi: 10.2307/1934981
Bazzaz, F., and Pickett, S. (1980). Physiological ecology of tropical succession: a comparative review. Annu. Rev. Ecol. Syst. 11, 287–310. doi: 10.1146/annurev.es.11.110180.001443
Beltrán, E., Verdú, M., and Valiente-Banuet, A. (2012). Trait divergence and indirect interactions allow facilitation of congeneric species. Ann. Bot. 110, 1369–1376. doi: 10.1093/aob/mcs089
Biber, P., Seifert, S., Zaplata, M. K., Schaaf, W., Pretsch, H., and Fischer, A. (2013). Relationships between substrate, surface characteristics, and vegetation in an initial ecosystem. Biogeosciences 10, 8283–8303. doi: 10.5194/bg-10-8283-2013
Blanchet, F. G., Legendre, P., and Borcard, D. (2008). Forward selection of explanatory variables. Ecology 89, 2623–2632. doi: 10.1890/07-0986.1
Bu, W., Zang, R., and Ding, Y. (2014). Functional diversity increases with species diversity along successional gradient in a secondary tropical lowland rainforest. Trop. Ecol. 55, 393–401.
Cadotte, M. W., Carscadden, K., and Mirotchnick, N. (2011). Beyond species: functional diversity and the maintenance of ecological processes and services. J. Appl. Ecol. 48, 1079–1087. doi: 10.1111/j.1365-2664.2011.02048.x
Chalmandrier, L., Munkemuller, T., Colace, M. P., Renaud, J., Aubert, S., Carlson, B. Z., et al. (2017). Spatial scale and intraspecific trait variability mediate assembly rules in alpine grasslands. J. Ecol. 105, 277–287. doi: 10.1111/1365-2745.12658
Chapin, I. I. I., Zavaleta, E. S., Eviner, V. T., Naylor, R. L., Vitousek, P. M., Reynolds, H. L., et al. (2000). Consequences of changing biodiversity. Nature 405, 234–242. doi: 10.1038/35012241
Chu, C. J., Maestre, F. T., Xiao, S., Weiner, J., Wang, Y. S., Duan, Z. H., et al. (2008). Balance between facilitation and resource competition determines biomass–density relationships in plant populations. Ecol. Lett. 11, 1189–1197. doi: 10.1111/j.1461-0248.2008.01228.x
Coomes, D. A., and Grubb, P. J. (2003). Colonization, tolerance, competition and seed-size variation within functional groups. Trends Ecol. Evol. 18, 283–291. doi: 10.1016/S0169-5347(03)00072-7
de Bello, F. (2012). The quest for trait convergence and divergence in community assembly: are null-models the magic wand? Glob. Ecol. Biogeogr. 21, 312–317. doi: 10.1111/j.1466-8238.2011.00682.x
de Bello, F., Price, J. N., Münkemüller, T., Liira, J., Zobel, M., Thuiller, W., et al. (2012). Functional species pool framework to test for biotic effects on community assembly. Ecology 93, 2263–2273. doi: 10.1890/11-1394.1
Diaz, S., Lavorel, S., McIntyre, S., Falczuk, V., Casanoves, F., Milchunas, D. G., et al. (2007). Plant trait responses to grazing–a global synthesis. Glob. Change Biol. 13, 313–341. doi: 10.1111/j.1365-2486.2006.01288.x
Donath, W. T., Holzel, N., and Otte, A. (2006). Influence of competition by sown grass, disturbance and litter on recruitment of rare flood-meadow species. Biol. Conserv. 130, 315–323. doi: 10.1016/j.biocon.2005.12.022
Duprè, C., Stevens, C. J., Ranke, T., Bleeker, A., Peppler-Lisbach, C., Gowing, D. J., et al. (2010). Changes in species richness and composition in European acidic grasslands over the past 70 years: the contribution of cumulative atmospheric nitrogen deposition. Glob. Change Biol. 16, 344–357. doi: 10.1111/j.1365-2486.2009.01982.x
Ellenberg, H. (1996). Vegetation Mittteleuropas mit den Alpen, 5th Edition. Stuttgart: Eugen Ulmer (in German).
Gallien, L., Zurell, D., and Zimmermann, N. E. (2018). Frequency and intensity of facilitation reveal opposing patterns along a stress gradient. Ecol. Evol. 8, 2171–2181. doi: 10.1002/ece3.3855
Gotelli, N. J., and McCabe, D. J. (2002). Species co-occurrence: a meta-analysis of JM Diamond's assembly rules model. Ecology 83, 2091–2096. doi: 10.1890/0012-9658(2002)083[2091:SCOAMA]2.0.CO;2
Granath, G., Strengbom, J., and Rydin, H. (2010). Rapid ecosystem shifts in peatlands: linking plant physiology and succession. Ecology 91, 3047–3056. doi: 10.1890/09-2267.1
Grime, J. P. (2006). Trait convergence and trait divergence in herbaceous plant communities: mechanisms and consequences. J. Veg. Sci. 17, 255–260. doi: 10.1111/j.1654-1103.2006.tb02444.x
Humbert, J. Y., Dwyer, J. M., Andrey, A., and Arlettaz, R. (2016). Impacts of nitrogen addition on plant biodiversity in mountain grasslands depend on dose, application duration and climate: a systematic review. Glob. Change Biol. 22, 110–120. doi: 10.1111/gcb.12986
Kembel, S. (2010). Picante: R tools for integrating phylogenies and ecology. Bioinformatics 26, 1463–1472. doi: 10.1093/bioinformatics/btq166
Klein, J. A., Harte, J., and Zhao, X. Q. (2004). Experimental warming causes large and rapid species loss, dampened by simulated grazing, on the Tibetan Plateau. Eco. Lett. 7, 1170–1179. doi: 10.1111/j.1461-0248.2004.00677.x
Kraft, N. J., and Ackerly, D. D. (2010). Functional trait and phylogenetic tests of community assembly across spatial scales in an Amazonian forest. Ecol. Monogr. 80, 401–422. doi: 10.1890/09-1672.1
Kunstler, G., Lavergne, S., Courbaud, B., Thuiller, W., Vieilledent, G., Zimmermann, N. E., et al. (2012). Competitive interactions between forest trees are driven by species' trait hierarchy, not phylogenetic or functional similarity: implications for forest community assembly. Ecol. Lett. 15, 831–840. doi: 10.1111/j.1461-0248.2012.01803.x
Legendre, P., Mi, X., Ren, H., Ma, K., Yu, M., Sun, I.-F., et al. (2009). Partitioning beta diversity in a subtropical broad-leaved forest of China. Ecology 90, 663–674. doi: 10.1890/07-1880.1
Levine, J. M., and Murrell, D. J. (2003). The community-level consequences of seed dispersal patterns. Annu. Rev. Ecol. Evol. Syst. 1, 549–574. doi: 10.1146/annurev.ecolsys.34.011802.132400
Li, X. L., Gao, J., Brierley, G., Qiao, Y. M., Zhang, J., and Yang, Y. W. (2013). Rangeland degradation on the Qinghai-Tibetan plateau: implications for rehabilitation. Land Degrad. Dev. 24, 72–80. doi: 10.1002/ldr.1108
Lin, Y., Berger, U., Grimm, V., and Ji, Q. R. (2012). Differences between symmetric and asymmetric facilitation matter: exploring the interplay between modes of positive and negative plant interactions. J. Ecol. 100, 1482–1491. doi: 10.1111/j.1365-2745.2012.02019.x
Liu, Y., Wu, G. L., Ding, L. M., Tian, F. P., and Shi, Z. H. (2017). Diversity-productivity trade-off during converting cropland to perennial grassland in the semi-arid areas of China. Land Degrad. Dev. 28, 699–707. doi: 10.1002/ldr.2561
Lohbeck, M., Poorter, L., Lebrija-Trejos, E., Martínez-Ramos, M., Meave, J. A., Paz, H., et al. (2013). Successional changes in functional composition contrast for dry and wet tropical forest. Ecology 94, 1211–1216. doi: 10.1890/12-1850.1
Lohbeck, M., Poorter, L., Martínez-Ramos, M., Rodriguez-Velázquez, J., Breugel, M., and Bongers, F. (2014). Changing drivers of species dominance during tropical forest succession. Funct. Ecol. 28, 1052–1058. doi: 10.1111/1365-2435.12240
Lohbeck, M., Poorter, L., Paz, H., Pla, L., van Breugel, M., Martínez-Ramos, M., et al. (2012). Functional diversity changes during tropical forest succession. Perspect. Plant Ecol. Evol. Syst. 14, 89–96. doi: 10.1016/j.ppees.2011.10.002
Lue, Y., Fu, B., Wei, W., Yu, X., and Sun, R. (2011). Major ecosystems in China: dynamics and challenges for sustainable management. Environ. Manage. 48, 13–27. doi: 10.1007/s00267-011-9684-6
Marcante, S., Winkler, E., and Erschbamer, B. (2009). Population dynamics along a primary succession gradient: do alpine species fit into demographic succession theory? Ann. Bot. 4, 211–216. doi: 10.1093/aob/mcp047
Mason, N. W., Richardson, S. J., Peltzer, D. A., de Bello, F., Wardle, D. A., and Allen, R. B. (2012). Changes in coexistence mechanisms along a long-term soil chronosequence revealed by functional trait diversity. J. Ecol. 100, 678–689. doi: 10.1111/j.1365-2745.2012.01965.x
Mayfield, M. M., and Levine, J. M. (2010). Opposing effects of competitive exclusion on the phylogenetic structure of communities. Ecol. Lett. 13, 1085–1093. doi: 10.1111/j.1461-0248.2010.01509.x
McGill, B. J., Enquist, B. J., Weiher, E., and Westoby, M. (2006). Rebuilding community ecology from functional traits. Trends Ecol. Evol. 21, 178–185. doi: 10.1016/j.tree.2006.02.002
Millennium Ecosystem Assessment (2005). Millennium Ecosystem Assessment, Ecosystems and Human Well-Being: Synthesis. Washington, DC: Island Press.
Myers, J. A., Chase, J. M., Jimenez, I., Jorgensen, P. M., Araujo-Murakami, A., Seidel, R., et al. (2013). Beta-diversity in temperate and tropical forests reflects dissimilar mechanisms of community assembly. Ecol. Lett. 16, 151–157. doi: 10.1111/ele.12021
Oksanen, J., Blanchet, F. G., Kindt, R., Legendre, P., Minchin, P. R., and O'Hara, R. B. (2016). Vegan: Community Ecology Package. R package version 2.4-0. Available online at: https://CRAN.~R-project (accessed April 4, 2019).
Orrock, J. L., and Christopher, C. C. (2010). Density of intraspecific competitors determines occurrence and benefits of accelerated germination. Am. J. Bot. 97, 694–699. doi: 10.3732/ajb.0900051
Pavoine, S., and Bonsall, M. B (2011). Measuring biodiversity to explain community assembly: a unified approach. Biol. Rev. 4, 792–912. doi: 10.1111/j.1469-185X.2010.00171.x
Petchey, O. L., and Gaston, K. J. (2006). Functional diversity: back to basics and looking forward. Ecol. Lett. 9, 741–758. doi: 10.1111/j.1461-0248.2006.00924.x
Poorter, L., de Plassche, M. V., Willems, S., and Boot, R. G. A. (2004). Leaf traits and herbivory rates of tropical tree species differing in successional status. Plant Biol. 6, 746–754. doi: 10.1055/s-2004-821269
Prach, K., and Walker, L. R. (2011). Four opportunities for studies of ecological succession. Trends Ecol. Evol. 26, 119–123. doi: 10.1016/j.tree.2010.12.007
Pulsford, S. A., Lindenmayer, D. B., and Driscoll, D. A. (2016). A succession of theories: purging redundancy from disturbance theory. Biol. Rev. 91, 148–167. doi: 10.1111/brv.12163
Ricotta, C., and Moretti, M. (2011). CWM and Rao's quadratic diversity: a unified framework for functional ecology. Oecologia 167, 181–188. doi: 10.1007/s00442-011-1965-5
Siefert, A. (2012). Spatial patterns of functional divergence in old-field plant communities. Oikos 121, 907–914. doi: 10.1111/j.1600-0706.2011.19706.x
Spasojevic, M. J., and Suding, K. N. (2012). Inferring community assembly mechanisms from functional diversity patterns: the importance of multiple assembly processes. J. Ecol. 100, 652–661. doi: 10.1111/j.1365-2745.2011.01945.x
Stephane, D. (2013). SpacemakeR: Spatial Modelling. R packageversion 0.0-5/r113. Available online at: http://R-Forge.R-project.org/projects/sedar/ (accessed April 4, 2019).
Stevens, C. J., Dise, N. B., Mountford, J. O., and Gowing, D. J. (2004). Impact of nitrogen deposition on the species richness of grasslands. Science 303, 1876–1879. doi: 10.1126/science.1094678
Tilman, D. (1987). Secondary succession and the pattern of plant dominance along experimental nitrogen gradients. Ecol. Monogra. 57, 189–214. doi: 10.2307/2937080
Tilman, D., Reich, P. B., Knops, J., Wedi, D., and Lehman, C. (2001). Diversity and productivity in a long-term grassland experiment. Science 294, 843–845. doi: 10.1126/science.1060391
Tóth, E., Deák, B., Valkó, O., Kelemen, A., Miglécz, T., Tóthmérész, B., et al. (2016). Livestock type is more crucial than grazing intensity: traditional cattle and sheep grazing in short-grass steppes. Land Degrad. Dev. 100, 52–61. doi: 10.1002/ldr.2514
Turnbull, L. A., Paul-Victor, C., Schmid, B., and Purves, D. W. (2008). Growth rates, seed size, and physiology: do small-seeded species really grow faster? Ecology 89, 1352–1363. doi: 10.1890/07-1531.1
Verdú, M., Rey, P. J., Alcántara, J. M., Siles, G., and Valiente-Banuet, A. (2009). Phylogenetic signatures of facilitation and competition in successional communities. J. Ecol. 97, 1171–1180. doi: 10.1111/j.1365-2745.2009.01565.x
Wang, C., Long, R., Wang, Q., Jing, Z., and Shi, J. (2009). Changes in plant diversity, biomass and soil C, in alpine meadows at different degradation stages in the headwater region of three rivers, China. Land Degrad. Dev. 20, 187–198. doi: 10.1002/ldr.879
Wang, W., Wang, Q., Wang, C. Y., Shi, H., Li, Y., and Wang, G. (2005). The effect of land d soils of alpine meadows on the Tibetan plateau. Land Degrad. Dev. 16, 405–415. doi: 10.1002/ldr.661
Xu, J., Michalet, R., Zhang, J. L., Wang, G., Chu, C. J., and Xiao, S. (2010). Assessing facilitative responses to a nurse shrub at the community level: the example of Potentilla fruticosa in a sub-alpine grassland of northwest China. Plant Biol. 12, 780–787. doi: 10.1111/j.1438-8677.2009.00271.x
Zaplata, M. K., Winter, S., Biemelt, D., and Fischer, A. (2011). Immediate shift towards source dynamics: the pioneer species Conyza canadensis in an initial ecosystem. Flora 206, 928–934. doi: 10.1016/j.flora.2011.07.001
Zaplata, M. K., Winter, S., Fischer, A., Kollmann, J., and Ulrich, W. (2013). Species-driven phases and increasing structure in early-successional plant communities. Am. Nat. 181, E17–E27. doi: 10.1086/668571
Zhang, H., Chen, H. Y. H., Lian, J., John, R., Ronghua, L., Liu, H., et al. (2018). Using functional trait diversity patterns to disentangle the scale-dependent ecological processes in a subtropical forest. Funct. Ecol. 32, 1379–1389. doi: 10.1111/1365-2435.13079
Zhang, H., Gilbert, B., Zhang, X., and Zhou, S. (2013). Community assembly along a successional gradient in sub-alpine meadows of the Qinghai-Tibetan Plateau, China. Oikos 122, 952–960. doi: 10.1111/j.1600-0706.2012.20828.x
Zhang, H., John, R., Peng, Z., Yuan, J., Chu, C., Du, G., et al. (2012). The Relationship between species richness and evenness in plant communities along a successional gradient: a study from sub-alpine meadows of the eastern Qinghai-Tibetan Plateau, China. PLoS ONE 7:e49024. doi: 10.1371/journal.pone.0049024
Zhang, H., Qi, W., John, R., Wang, W. B., Song, F. F., and Zhou, S. R. (2015). Using functional trait diversity to evaluate the contribution of multiple ecological processes to community assembly during succession. Ecography 38, 11–21. doi: 10.1111/ecog.01123
Keywords: abiotic filtering, chronosequence, functional trait diversity-based tests, hierarchical competition, interspecific facilitation, secondary succession, niche-based competition, spatial structure
Citation: Zhang H, John R, Liu K, Qi W and Long W (2019) Using Functional Trait Diversity Patterns to Disentangle the Processes Influencing the Recovery of Subalpine Grasslands Following Abandonment of Agricultural Use. Front. Ecol. Evol. 7:128. doi: 10.3389/fevo.2019.00128
Received: 14 November 2018; Accepted: 29 March 2019;
Published: 16 April 2019.
Edited by:
Urs Feller, University of Bern, SwitzerlandReviewed by:
Isabela Galarda Varassin, Federal University of Paraná, BrazilGerhard Karrer, University of Natural Resources and Life Sciences Vienna, Austria
Markus Klemens Zaplata, Nagola Re GmbH, Germany
Copyright © 2019 Zhang, John, Liu, Qi and Long. This is an open-access article distributed under the terms of the Creative Commons Attribution License (CC BY). The use, distribution or reproduction in other forums is permitted, provided the original author(s) and the copyright owner(s) are credited and that the original publication in this journal is cited, in accordance with accepted academic practice. No use, distribution or reproduction is permitted which does not comply with these terms.
*Correspondence: Hui Zhang, zhanghuitianxia@163.com
WenXing Long, oklong@hainu.edu.cn