The Role of Vessel Biofouling in the Translocation of Marine Pathogens: Management Considerations and Challenges
- 1Ministry for Primary Industries, Wellington, New Zealand
- 2Marine Invasive Species Program, California State Lands Commission, Long Beach, CA, United States
- 3Cawthron Institute, Nelson, New Zealand
- 4Chesapeake Biological Laboratory, University of Maryland Center for Environmental Science, Solomons, MD, United States
- 5U.S. Naval Research Laboratory, Washington, DC, United States
- 6Smithsonian Environmental Research Center, Edgewater, MD, United States
- 7Invasive Species Branch, Biosecurity Tasmania, Department of Primary Industries, Parks, Water and Environment, Hobart, TAS, Australia
- 8SARDI Aquatic Sciences and Marine Innovation Southern Australia, South Australian Research and Development Institute, West Beach, SA, Australia
Vessel biofouling is a major pathway for the introduction, establishment, and subsequent spread of marine non-indigenous macro-organisms. As a result, national and international regulations and guidelines have been implemented to manage the risks associated with this pathway, yet widespread enforcement and uptake are still in their infancy. By comparison, translocation of marine pathogens by vessel biofouling has received little attention despite a mounting body of evidence highlighting the potential importance of this pathway. Using molluscan pathogens as a model, this paper examines the potential for translocation of marine pathogens via the vessel biofouling pathway by reviewing: (1) examples where vessel biofouling is suspected to be the source pathway of non-indigenous pathogen introduction to new areas, and (2) the association between pathogens known to have detrimental effects on wild and farmed mollusk populations with species known to foul vessels and anthropogenic structures. The available evidence indicates that vessel biofouling is a viable and important pathway for translocating marine pathogens, presenting a risk to marine values (i.e., environmental, economic, social, and cultural). While preventive measures to minimize the translocation of macro-organisms are the most efficient way to minimize the likelihood of associated pathogen translocation, the application of reactive management measures to biofouled vessels, including post-filtration treatment, requires further and explicit consideration.
Introduction
The International Maritime Organization (IMO) defines biofouling as the growth and accumulation of organisms on immersed ship surfaces or structures (International Maritime Organization [IMO], 2011). Typically, any substrate placed in natural waters is quickly colonized by micro-organisms (creating a biofilm, also known as the slime layer) that is followed by a succession of diverse sessile or sedentary micro- and macro-organisms (Flemming, 2002; Aldred and Clare, 2008; Amara et al., 2018). Vessel biofouling is acknowledged as a major, and perhaps the most important, pathway for the introduction, establishment, and subsequent spread of marine non-indigenous macro-organisms (Drake and Lodge, 2007; Hewitt and Campbell, 2010; Bell et al., 2011; Ruiz et al., 2015; Bailey et al., 2020). Similar to the concerns over the transport of human pathogens in ships’ ballast water (McCarthy and Khambaty, 1994; Ruiz et al., 2000a; Cohen et al., 2012), the potential for vessel biofouling to act as a vector for non-indigenous pathogens has been highlighted for some time (e.g., Howard, 1994). Pathogens have been found in biofilms growing on vessel surfaces (e.g., Drake et al., 2007; Shikuma and Hadfield, 2010), and mature organisms within vessel biofouling assemblages are more likely to harbor pathogens than their younger or larval planktonic stages associated with ballast water (Hine, 1995). For the purpose of this document, the term pathogen is used to include viruses, bacteria, protists, and fungi that cause disease in other organisms; the term vessel is used to include every description of ship, boat, or other craft used in water navigation, i.e., both recreational and commercial vessels are included.
The role of anthropogenic vectors in global scale biotic exchange is largely based on patterns and processes linked to macro-organism translocations and biogeography (Ruiz et al., 2000b; Pagenkopp-Lohan et al., 2020), thus the magnitude and impacts of marine micro-organism translocations are likely “vastly underestimated” (Pagenkopp-Lohan et al., 2020). Practical difficulties in micro-organism identification and detection, and a lack of baseline knowledge about native organism geographical ranges, however, have hampered research efforts (Davidson et al., 2013; Pagenkopp-Lohan et al., 2020).
In the risk analysis to support implementation of New Zealand’s biofouling regulations (Ministry for Primary Industries New Zealand [MPI], 2018), Bell et al. (2011) identified recent changes to shipping patterns including increased shipping volumes, expansion of trade routes, and increased vessel speeds were providing a greater likelihood of translocation of marine non-indigenous macro-organisms. The delivery rate of micro-organisms associated with vessel biofouling may be similarly increasing over time (Pagenkopp-Lohan et al., 2020). The role of shipping, particularly vessel biofouling, in pathogen translocations may, therefore, undermine regulations and improved management practices aimed at addressing the major anthropogenic vectors for historical pathogen translocations, such as aquaculture and fisheries stocking (Williams et al., 2013; Georgiades et al., 2016; Pagenkopp-Lohan et al., 2020). While vessel biofouling has also been subject to increased scrutiny and management in some jurisdictions (Georgiades et al., 2020; Scianni et al., in press), it remains a largely unregulated broad-scale vector of organisms both domestically and internationally.
The threats posed by non-indigenous pathogens are similar to marine non-indigenous macro-organisms: once established they are difficult to control, and eradication is often infeasible or unsuccessful (Centre for Environment, Fisheries and Aquaculture Science [CEFAS], 2009; Georgiades et al., 2020). Prevention is therefore the only effective measure (Centre for Environment, Fisheries and Aquaculture Science [CEFAS], 2009; Georgiades et al., 2016). This is particularly the case for shellfish aquaculture and fisheries, where the introduction and establishment of novel pathogens can have devastating effects. For example, Bonamia ostreae and Marteilia refringens drastically reduced European production of cultured flat oysters (Ostrea edulis) from 29,595 t in 1961 to 5,921 t in 2000 (Culloty and Mulcahy, 2007). Between 1980 and 1983 alone, estimated losses in France included a 20% reduction of employment within the industry, US$ 240 million turn-over, and US$ 200 million of added value [Meuriot and Grizel (1984) in Arzul et al., 2006]. European flat oyster production has stabilized but at low levels (< 3,000 t; Goulletquer, 2004), using modified husbandry techniques with lower employment than the industry had historically provided (Arzul et al., 2006; Culloty and Mulcahy, 2007). Based on these impacts, introduction of B. ostreae to New Zealand in 2015 led to the pre-emptive depopulation of all farmed flat oyster (O. chilensis) stock to protect uninfected areas, particularly the Bluff wild oyster fishery at the southern tip of the South Island (Farnsworth et al., 2020).
The ostreid herpes virus microvariant 1 (OsHV-1) has caused mass mortalities in spat and juvenile Pacific oysters (Crassostrea gigas) in France (Ségarra et al., 2010), Australia (Paul-Pont et al., 2014), and New Zealand (Bingham et al., 2013). During initial outbreaks, stock losses of up to 100% were recorded (Keeling et al., 2014; European Food Safety Authority [EFSA], 2015), and the disease halved New Zealand’s Pacific oyster production (Johnston, 2014). The immediate impacts to industry and biosecurity response costs for OsHV-1 and B. ostreae in New Zealand have far outweighed those related to marine non-indigenous macro-organisms (Georgiades et al., 2020).
The introduction of Haplosporidium nelsoni to the mid-Atlantic coast of the United States in the 1950s also had extensive impacts on Crassostrea virginica populations, with mortality exceeding 90% in Delaware and Chesapeake Bays (Haskin and Ford, 1982; Haskin and Andrews, 1988). Between 1958 and 1983, it was estimated that H. nelsoni had reduced oyster landings in Delaware Bay by two-thirds (Haskin and Ford, 1982). The introduction of H. nelsoni, combined with pollution and oyster overharvesting, drove large-scale ecological impacts on Chesapeake Bay (Kemp et al., 2005), and extensive management and restoration efforts have achieved relatively modest success (National Research Council., 2004). The fishery in Chesapeake Bay declined to 2% of its historical catch in 30 years, and introduction and culture of non-native oyster species (including C. gigas and C. ariakensis) was seriously considered (Mann et al., 1991; Calvo et al., 1999; Tamburri et al., 2008).
While vessel biofouling regulations have been enacted by some jurisdictions (California Code of Regulations, 2017; Ministry for Primary Industries New Zealand [MPI], 2018; Georgiades et al., 2020), these are focused largely on preventing the translocation of marine non-indigenous macro-organisms (e.g., Bell et al., 2011). New information on translocation of pathogens associated with biofouling is emerging, however (e.g., Lane et al., 2018; Itoh et al., 2019; Costello et al., 2020; Lane and Jones, 2020; Pagenkopp-Lohan et al., 2020). Biofouling management measures routinely applied to vessels, such as in-water cleaning of macrofouling [i.e., reactive in-water cleaning (RIC)], are also cause for concern as they may increase the likelihood of pathogen release and establishment into new areas (Scianni and Georgiades, 2019).
In light of the major negative consequences novel pathogen introductions have thus far caused, understanding the risk of pathogen translocation by vessel biofouling is critical to inform biosecurity guidelines, regulations, and management approaches to protect marine values such as biodiversity, customary and recreational practices, fisheries, and aquaculture. This analysis reviews the literature to investigate the likelihood of this translocation pathway, including the ramifications for vessel maintenance, and discusses potential risk management options, as appropriate.
Analysis
Analysis Scope
This analysis primarily focuses on the pathogens of mollusks as a model. There are numerous World Organization for Animal Health (OIE) listed or emerging molluscan pathogens that are of major concern for jurisdictions worldwide (Bower, 2017; OIE, 2020). The implications, conclusions, and recommendations drawn from the molluscan model may have broad application to other marine and human pathogens.
Pathogen Translocation Associated With Vessel Biofouling
Howard (1994) noted that the domestic transfer of B. ostreae from Cornwall to Plymouth (England) likely occurred with biofouling, which included live mollusks, on concrete barges. This conclusion was based on Plymouth having no history of the disease nor any reason to receive live oyster transfers for aquaculture purposes (Howard, 1994). The spread of B. ostreae has also been linked to vessel biofouling in the Netherlands (van Banning, 1991) and Ireland (Culloty and Mulcahy, 2007).
Bonamia ostreae was detected in the Southern Hemisphere (New Zealand) in 2015 (Georgiades, 2015; Lane et al., 2016) and was most likely introduced by vessel biofouling (Lane et al., 2020). The spread of B. exitiosa, from southern New Zealand to the Northern Hemisphere (Bishop et al., 2006; Abollo et al., 2008; Longshaw et al., 2013) and Argentina (Kroeck and Montes, 2005), has similarly been associated with shipping (Hill-Spanik et al., 2015; Lane et al., 2018). B. ostreae and other molluscan pathogens are associated with non-indigenous ascidians (Costello et al., 2020), highlighting the potential role of non-molluscan biofouling species as vectors of this pathogen.
Vessel biofouling is suggested as responsible for the introduction of OsHV-1 into New Zealand (Lane et al., 2020) and Australia (Fisheries Research and Development Corporation, 2011; Whittington et al., 2018), and its subsequent Australian spread to Tasmania and South Australia (Deveney et al., 2017). Fuhrmann and Hick (2020) found that laboratory transmission of OsHV-1 between donor and naive Pacific oysters via a simulated biofouling scenario was plausible but complex. While transmission from other biofouling species was not observed by Fuhrmann and Hick (2020), the association of OsHV-1 with some biofouling organisms (i.e., bryozoan species) suggested that they may protect the virus from degradation (Martenot et al., 2015; Hick et al., 2016). OsHV-1 transmission to naive Pacific oysters has also been shown following cohabitation with exposed wild crabs (Carcinus maenas; Bookelaar et al., 2018) and mussels (Mytilus spp.; O’Reilly et al., 2018). These taxa have previously been identified within vessel biofouling assemblages (Visscher, 1928; Apte et al., 2000; Moshchenko and Zvyagintsev, 2001; Coutts et al., 2003). The mechanisms by which marine non-indigenous species can affect pathogen-host interactions are complex (Goedknegt et al., 2016), which has possible implications for their association and transport by vessels.
Vessel movements have also been linked to the introduction of H. nelsoni to the United States and Canada either through biofouling or release of H. nelsoni spores by ballast water discharges (National Research Council., 2004; Stephenson and Petrie, 2005). Hine (1996) highlighted the biofouling pathway as a risk for spreading Perkinsus marinus, and Pagenkopp-Lohan et al. (2018) and Itoh et al. (2019) further demonstrated the potential for shipping to contribute to the long-range dispersal of Perkinsus species.
In addition to pathogens, it is also noteworthy that parasitic invertebrates may be translocated by vessel biofouling (Davidson et al., 2013). Parasitic invertebrates were found to infect 7.8% of mussels sampled from 23 vessels operating on the U.S. West Coast, including the parasitic copepods Pseudomyicola spinosus and Modiolicola gracilis. Transport of infected mussels by international shipping has also been implicated in the intercontinental spread of a molluscan transmissible neoplasia (Yonemitsu et al., 2019).
In assessing pathogen risks associated with translocation of mollusk shells for reef restoration, Diggles (2020) noted the potential of molluscan pathogens, including iridoviruses, OsHV-1, and Bonamia species, to be introduced to Australia by vessel biofouling specifically and others, including H. nelsoni and Perkinsus species, by shipping more generally. The Australian Government Field Identification Guide for Aquatic Animal Diseases (Department of Agriculture, Water and the Environment, 2020) also recognizes vessel biofouling as a potential pathway for translocating various Bonamia species and OsHV-1. These examples show that the role of shipping, and more specifically vessel biofouling, in pathogen translocation is increasingly recognized as a serious risk factor for increasing the incidence of infection and disease outbreaks at various scales (within regions and over long-distance oceanic scales).
Associations of OIE-Listed and Other Important Pathogens With Known Biofouling Species
The OIE is an intergovernmental organization established to promote global animal health (OIE, 2019). To facilitate health certification and reduce risk in the trade of aquatic animals and their products, the OIE Aquatic Animal Health Standards Commission compiles the Manual of Diagnostic Tests for Aquatic Animals (the Aquatic Manual), records species known to be susceptible to listed pathogens, and provides standardized, validated approaches for diagnosis.
The chapters of the OIE Aquatic Manual (OIE, 2019) specific to molluscan pathogens show that many known susceptible species are associated with biofouling of vessels, anthropogenic structures within harbors, hard substrates, or offshore installations (Table 1). Further, many of these pathogens either have poorly understood life cycles (e.g., B. exitiosa) or have been shown to survive outside the host for periods of weeks to months (e.g., B. ostreae, P. marinus, and P. olseni; OIE, 2019). There are also many molluscan pathogens that, although not listed by the OIE, cause substantial impacts and are associated with biofouling species (Table 2).
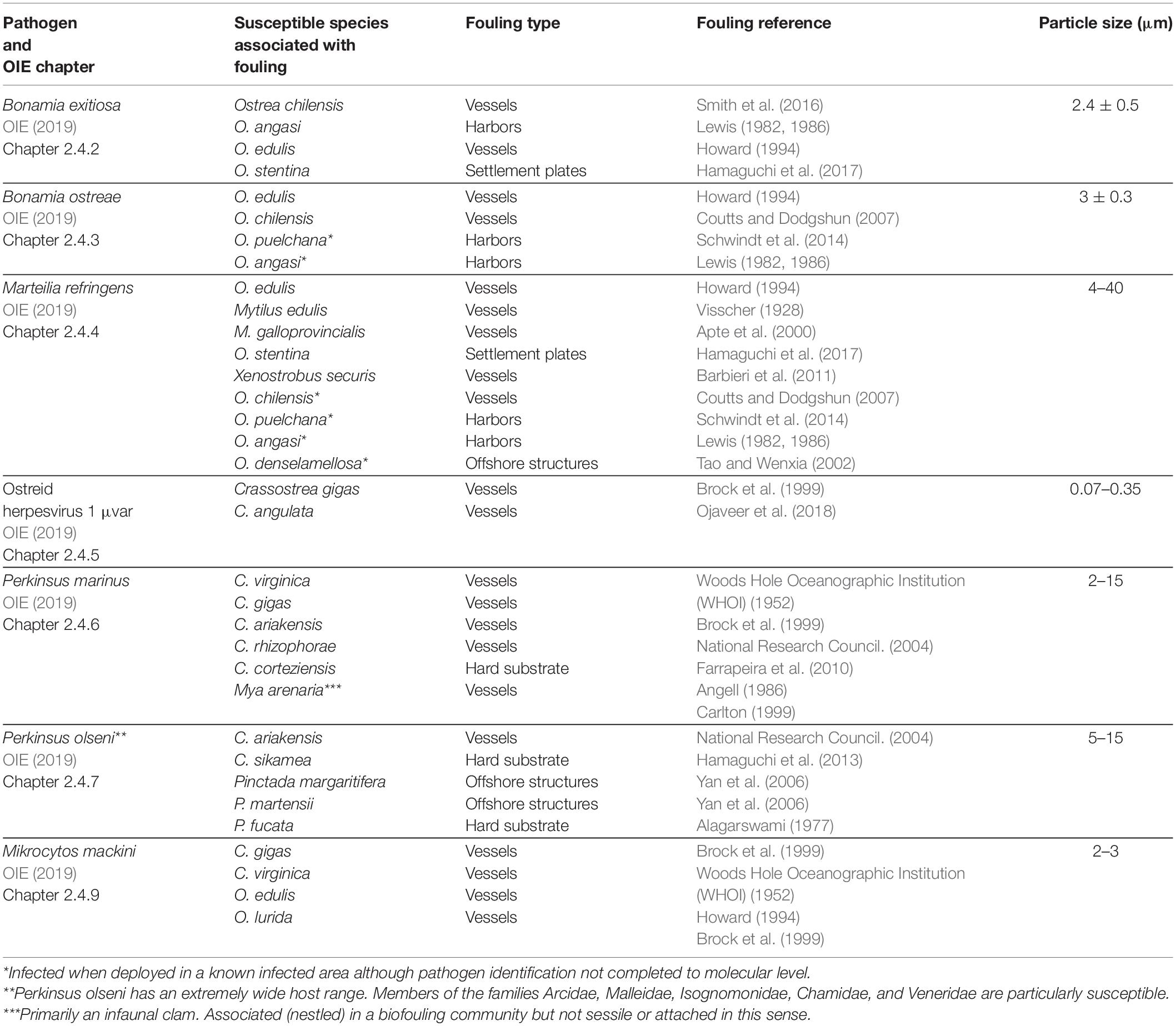
Table 1. OIE-listed molluscan pathogens associated with known fouling species and their size (Bower, 2017; OIE, 2019).
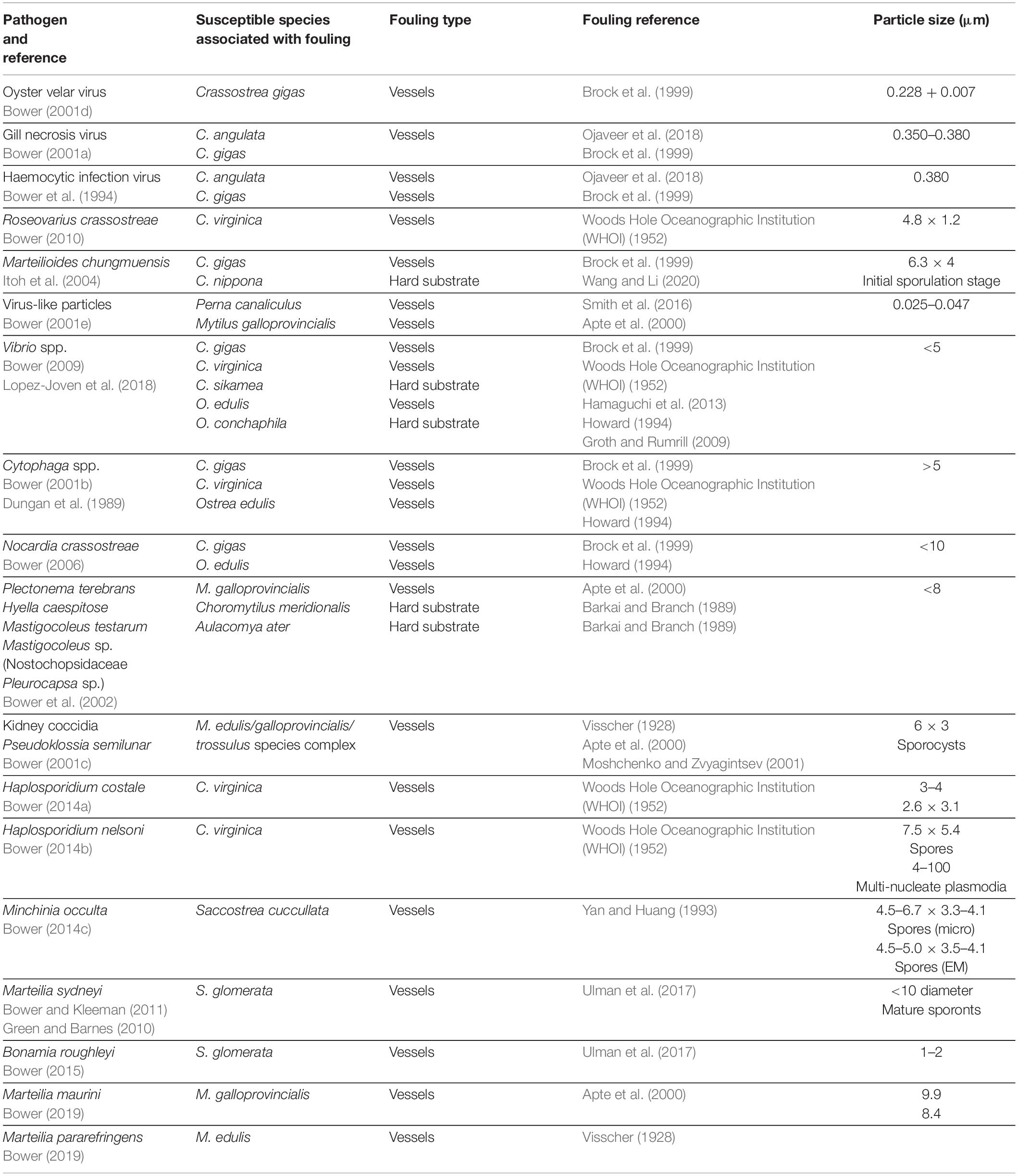
Table 2. Examples of non-OIE listed molluscan pathogens that have caused substantial impacts and are associated with known fouling species (per Bower, 2017).
Pathogen translocation via the biofouling pathway adds further layers of complexity to factors that influence pathogen transmission and disease dynamics (Fuhrmann and Hick, 2020; Lane et al., 2020). These parameters include pathogen life cycles, host specificity and susceptibility, infective dose, survival outside host, and susceptible host life stages (OIE, 2019; Pagenkopp-Lohan et al., 2020). To understand the translocation dynamics of these pathogens, presence of host or carrier species on vessel submerged surfaces, exposure of those species to pathogens prior to transit, prevalence and intensity of infection, and time of year (i.e., both seasonal dynamics and environmental conditions encountered) need to be considered.
Introduction of pathogens to new environments is influenced by vessel itinerary (i.e., places visited and duration of stay) and the type and duration of exposure of hosts in recipient environments. For example, exposure may occur as a result of pathogen shedding from organisms on a vessel, release of macro-organisms from the vessel surface, or an uncontained release of biofouling and pathogens following in-water cleaning. Characteristics of the recipient environment also need to be considered, including temperature, salinity, pollution, and—importantly—the presence, proximity, and density of susceptible host or carrier species (OIE, 2019; Lane et al., 2020; Pagenkopp-Lohan et al., 2020).
Receiving environments for vessels are typically ports and marinas which, being heavily modified, offer a variety of habitats for colonization by sessile and mobile taxa, including non-indigenous species (Ruiz et al., 1997; Johnston et al., 2017). These environments are often enclosed, leading to high particle retention (Gadd et al., 2011; Morrisey et al., 2013), thus, if released, pathogens may stay in contact with host species that are present for longer periods at potentially infective doses. The presence of artificial and modified habitats, combined with relatively high retention, enhances conditions for introduction, establishment, and spread of new non-indigenous macro-organisms (Floerl et al., 2009; Ruiz et al., 2009; Johnston et al., 2017) and associated pathogens. Following arrival and colonization, domestic vessels provide a vital link for spread from primary infected areas via movement of associated ballast water (Inglis et al., 2013) and biofouling, including fouled vessels that transit aquaculture zones (Sim-Smith et al., 2016).
Not every mollusk that is translocated with vessel fouling will cause a novel pathogen to establish with subsequent consequences (e.g., Gias and Johnston, 2010; Davidson et al., 2013; Fuhrmann and Hick, 2020). Mounting evidence from laboratory and field observations as well as documented consequences, however, indicates that the risks associated with this pathway are non-negligible and that risk management measures may be justified. The evidence chain outlined here is consistent with the criteria applied to assess the risks associated with vessel biofouling for marine non-indigenous macro-organism translocations (Bell et al., 2011), which was a key step that led to biofouling regulations in New Zealand (Georgiades et al., 2020).
Mollusks are, importantly, not the only taxa associated with vessel biofouling that may translocate pathogens of concern. The invasive crabs Eriocheir sinensis and C. maenas are associated with fouled vessels (Peters and Panning, 1933 in Herborg et al., 2003; Coutts et al., 2003) and are susceptible hosts or carriers of several pathogens with well-documented consequences to marine values, including fisheries and aquaculture (Table 3). Parasitic invertebrates can also be translocated by barnacles, including the castrating isopod Hemioniscus balani (Davidson et al., 2013). Human pathogens, such as Vibrio cholerae, Vibrio parahaemolyticus, and Escherichia coli, have also been found in the surface biofilms of vessels (Shikuma and Hadfield, 2010; Revilla-Castellanos et al., 2015).
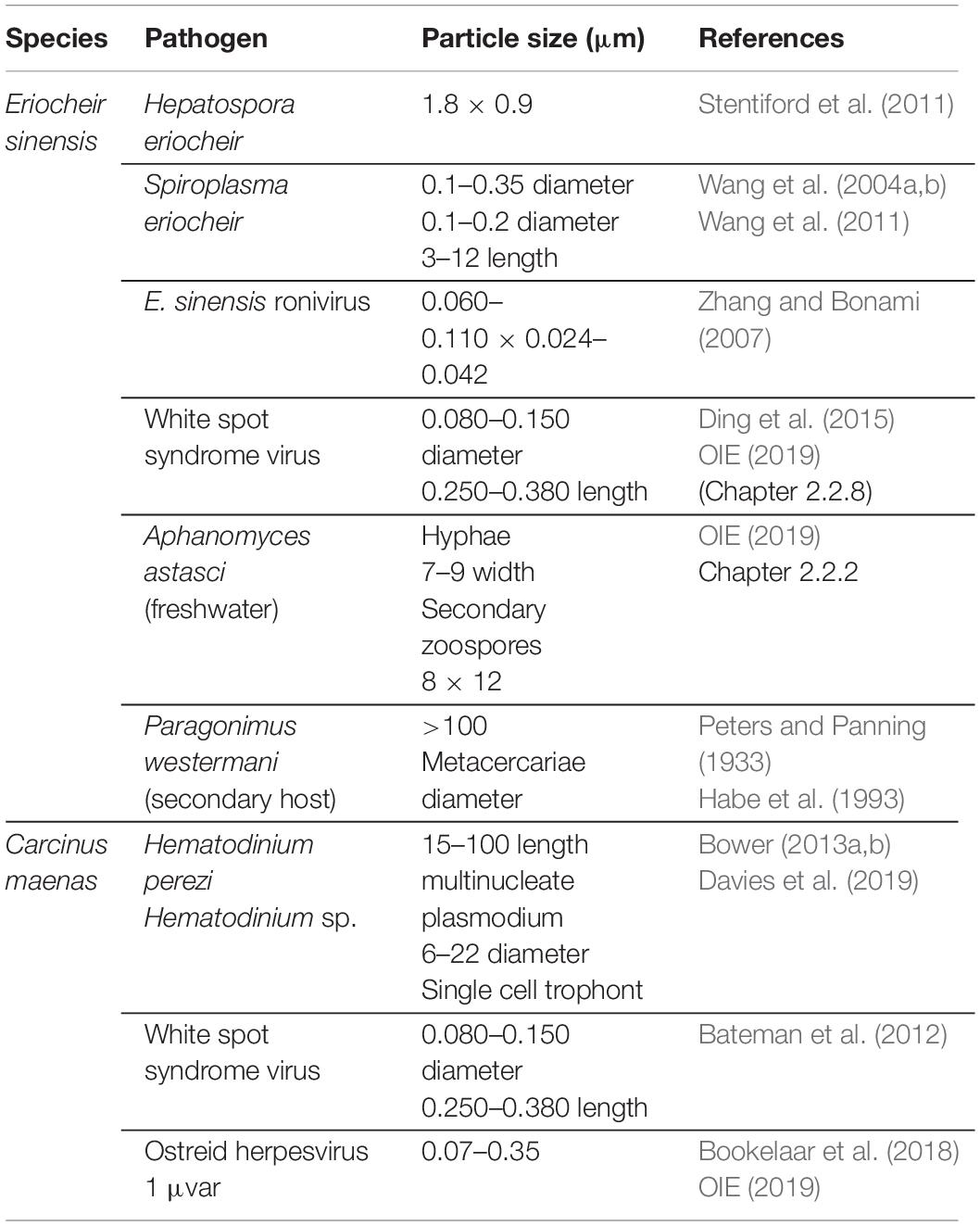
Table 3. Notable pathogens and parasitic invertebrates associated with Eriocheir sinensis and Carcinus maenas.
Potential Management Options
Pathogens can be released from vessel biofouling by being: (a) sloughed from the attached biofilm, (b) dispersed by proactive in-water cleaning (PIC), (c) shed from macrofouling that remains intact on the vessel, (d) shed with macrofouling released during normal vessel operations (i.e., drop-off of attached species or active escape of mobile species), or (e) dispersed with or without their hosts during application of RIC. We have identified a two-pronged approach to protect marine values from pathogen introductions associated with vessel biofouling by: (1) limiting the volume and frequency of pathogen translocations via ongoing vessel transportation (i.e., propagule pressure; Lockwood et al., 2005) and (2) avoiding pathogen releases by reactive management activities. The methods used to achieve these approaches all have associated advantages and disadvantages (Table 4).
The maritime antifouling industry is established to prevent and manage biofouling on vessels. The primary focus has been on surface paints or coatings on the immersed surfaces of ships to prevent macrofouling growth (using biocides, such as copper- and zinc-based compounds) and/or restrict macrofouling adhesion (non-biocidal or fouling-release, such as silicone-based coatings; Dafforn et al., 2011; Lewis, 2020). The use of biocidal coatings represents a trade-off between vessel fuel efficiency, reduced exhaust emissions, and reduced translocation of non-indigenous species, versus environmental impacts of the biocides (Dafforn et al., 2011; Scianni and Georgiades, 2019; Richir et al., 2021). Even though these coatings have evolved toward more sophisticated, cost effective, and environmentally acceptable products, antifoulants do not prevent biofilm formation (Dobretsov, 2010) or incidental macrofouling that establishes during vessel in-service periods (i.e., the time between vessel dry-docking; Georgiades and Kluza, 2017). There are areas of ships that cannot be painted (e.g., anodes), are difficult to paint (e.g., dry-dock support strips), or experience sub-optimal coating performance because of surface or hydrodynamic issues (e.g., sea chests, gratings, rudders, and projections). These “niche areas” are hotspots of biofouling accumulation (Coutts and Taylor, 2004; Davidson et al., 2009, 2016) that require ongoing vigilance and maintenance (California Code of Regulations, 2017; Georgiades et al., 2018; Ministry for Primary Industries New Zealand [MPI], 2018). While biocide release rates from coatings can be estimated and environmental concentrations predicted (e.g., Morrisey et al., 2013), no quantitative assessments or estimates have been made of the release rates or quantities of live micro- or macrofouling organisms into coastal ecosystems as a result of normal vessel operations.
In-water cleaning has emerged as the principal approach to address limitations in coating performance and operational impacts of biofouling that accumulate while in-service. In-water cleaning typically involves use of diver or remotely operated cleaning or cart systems that remove biofouling from hull surfaces (McClay et al., 2015; Morrisey and Woods, 2015; Tamburri et al., 2020). While in-water cleaning is often performed in response to fundamental operational factors, such as increasing fuel consumption (to reset hull surfaces to a more hydrodynamic state), it can have unintended consequences including: (a) increased release of antifouling biocides to ambient waters; (b) active liberation of live biofouling organisms or their propagules into local habitats, with increased risk of non-indigenous species introduction; and (c) diminished coating condition that reduces antifouling performance and longevity (Scianni and Georgiades, 2019; Tamburri et al., 2020). There is growing consensus internationally that steps should be taken to minimize these environmental impacts, by moving away from in-water cleaning of macrofouling that does not attempt to capture debris (i.e., RIC), and toward RIC that capture, contain, and treat debris (RICC), or PIC to prevent macrofouling establishment and growth. For all in-water cleaning systems, there are two main processes that can release biological material including pathogens: (a) application of the cleaning unit to the vessel surface (either through incomplete or ineffective capture of debris by the cleaning head) and (b) release of untreated or incompletely treated effluent (Scianni and Georgiades, 2019).
Pathway Management Approach
Few jurisdictions have enacted biofouling regulations to limit the translocation of marine non-indigenous macro-organisms (Georgiades et al., 2020; Scianni et al., in press). New Zealand’s Craft Risk Management Standard for Biofouling on Vessels Arriving to New Zealand (CRMS-BIOFOUL) defines “clean hull” thresholds which are governed by the vessel’s itinerary (Ministry for Primary Industries New Zealand [MPI], 2018). These thresholds, while acknowledging issues of feasibility and practicality, were designed to limit macro-organism species richness and density, constraining reproduction, and limiting establishment (Georgiades and Kluza, 2017). The holistic “level of fouling” approach applied by New Zealand manages risk but avoids difficulties, costs, and time associated with taxonomic identifications (Bell et al., 2011). This approach also protects against species not yet known to be invasive and does not require formation and ongoing maintenance of lists of “risk species” or the ongoing cost of surveillance and reporting against such lists.
The likelihood of pathogen translocation from vessels is most efficiently reduced by limiting the amount of macrofouling, which includes susceptible hosts and carrier species, on incoming international or inter-regional vessels. Similar to predicting invasive marine macro-organisms (Bell et al., 2011), identifying future high-risk marine pathogens is difficult; further, the relative dearth of information in this area (Lane et al., 2020; Pagenkopp-Lohan et al., 2020) and the complexities related to pathogen introduction and establishment (section “Associations of OIE-Listed and Other Important Pathogens With Known Biofouling Species”) create challenges and uncertainty in determining risk. A pathway management approach to holistically manage pathogen translocations associated with vessel biofouling is therefore likely to be more effective than a pathogen-specific approach.
In-water cleaning plays an important role in managing risks associated with the vessel biofouling pathway (Georgiades et al., 2018; Scianni et al., in press). There are, however, multiple approaches to consider in advancing a “cleaning strategy” to explicitly minimize the transfer of pathogens and micro-organisms. The following sections build on existing tools while identifying challenges, knowledge gaps, and possible solutions.
Proactive In-Water Cleaning (PIC) to Support Ongoing Vessel Maintenance
Proactive in-water cleaning (PIC) is an emerging approach used to prevent biofilm formation, to remove it from the hull (including microscopic life stages of macrofouling organisms), and ultimately to prevent or reduce the establishment and growth of macrofouling (Scianni and Georgiades, 2019). Because of its application early in the biofouling process, less abrasive techniques that are more consistent with the recommendations of antifouling system manufacturers may be used. While a substantial amount of microscopic material is released into the marine environment, PIC is viewed as a relatively low-risk activity because it minimizes the translocation of macrofouling species (Department of Agriculture [DOA] et al., 2015) and therefore minimizes potential translocation of pathogens replicating in macrofouling organisms (Hine, 1995; Lallias et al., 2008; Fuhrmann and Hick, 2020).
Harmful microalgae (including diatoms and dinoflagellates) and pathogens can occur in the biofilm of vessels (Drake et al., 2005, 2007; Molino and Wetherbee, 2008; Shikuma and Hadfield, 2010; Revilla-Castellanos et al., 2015). Biofilm formation and its subsequent sloughing from submerged vessel areas during normal operations (including interactions with tugs, bunkering barges, pilot boats, fenders, lines, etc.), however, cannot be prevented (Dobretsov, 2010; Morrisey et al., 2013) without near continual maintenance (Tribou and Swain, 2017; Amara et al., 2018). It may be conceivable to treat liberated biofilm associated micro-organisms during PIC [e.g., exposing the cleaning unit effluent to ultraviolet (UV) radiation], however, the role of the biofilm in pathogen and non-indigenous species translocations requires clarification, thus decisions about the utility and efficacy of PIC require consideration of this uncertainty.
The “Clean Before You Leave” Approach
To further limit the potential for pathogen translocation, adopting the reactive in-water cleaning (RIC) approach of “clean before you leave” could be especially useful, considering that the geographic origin of accumulated fouling dictates the biosecurity risk (Department of Agriculture [DOA] et al., 2015). This approach recognizes that the most effective focal point for management is prevention, and thus looks to manage the risk of pathogen translocation at the source to limit spread and downstream impacts (Ricciardi et al., 2020). The applicability of this practice depends on the vessel’s prior itinerary, pathogen status of the recipient area and areas visited earlier, and proximity to high-value areas. Management of environmental contamination by antifouling biocides is a key consideration if such an approach is to be used (Scianni and Georgiades, 2019).
Reactive In-Water Cleaning With Capture (RICC)
Reactive in-water cleaning of vessel biofouling includes methods to capture, contain, and treat associated organisms [i.e., RICC, also referred to as in-water cleaning with capture (IWCC)]. The efficacy of RICC systems is uncertain in many cases and may vary by organism size and local conditions (Davidson et al., 2008; Scianni and Georgiades, 2019; Tamburri et al., 2020). To date, cleaning units are designed primarily to capture macrofouling organisms, with some attention to treatment of chemical effluent (Scianni and Georgiades, 2019). Vessel surveys to assess if RICC of macro-organisms is required are limited to detection and identification of macrofouling, while pathogens that may be associated with biofouling organisms have seldom been considered (Georgiades et al., 2020).
Removal of macrofouling by RICC is likely to kill the hosts and, where appropriate treatment of waste is not applied, release pathogens if present. Infected mollusks that are dead or moribund are known pathogen sources, for example: OsHV-1 (see Sauvage et al., 2009), P. olseni (see Raynard et al., 2007), and P. marinus (see Bobo et al., 1997). The reactive removal of macrofouling can release infective material, potentially in large amounts, into receiving environments with high particle retention. A single oyster can contain 4.4 × 108 B. ostreae parasites (Lallias et al., 2008), and Arzul et al. (2009) observed 58% survival of B. ostreae parasites after 7 days exposure to seawater at 15°C. OsHV-1 has been observed at 107 DNA copies per mg of clinically affected Pacific oyster tissue (Pepin et al., 2008). Hick et al. (2016) found that OsHV-1 remained infectious in seawater for 2 days at 20°C and in non-viable oyster tissues (wet or dry) for at least 7 days at 20°C. Vigneron et al. (2004) detected OsHV-1 DNA released into seawater from macerated larvae for 22 days at 4°C and 12 days at 20°C, although it was not determined if this DNA was viable. P. olseni loads in infected host tissues can exceed 2 × 106 parasites per gram (Choi and Park, 2010), and P. olseni can survive outside a host for at least several months (Casas et al., 2002). All life stages of P. olseni are considered infective (Villalba et al., 2004). While elevated concentrations of antifouling biocides in the treatment stream (Tamburri et al., 2020) may render some pathogens non-viable (Dupont et al., 2011), these can also impact the health of organisms in the receiving environment (Dafforn et al., 2011; Amara et al., 2018), and potentially increase their susceptibility to disease (Moreau et al., 2015).
Direct releases of biofouling from the cleaning head can be assessed by analyzing the total suspended solids (TSS) in water sampled from the surrounding environment during equipment operation (Alliance for Coastal Technologies Maritime Environmental Resource Center [ACT/MERC], 2019; Tamburri et al., 2020). Inclusion of this analysis is a useful addition to the technical advice released by MPI on testing in-water cleaning and treatment systems for external hull and niche areas (Morrisey et al., 2015) and internal seawater systems (Growcott et al., 2019). Similar to direct video observations of the cleaning unit during operations, TSS may serve as a proxy for propagule release into the marine environment.
Recommendations for biosecure effluent treatment standards for surface-based waste processing systems associated with RICC are typically based on physical separation (typically settling tanks followed by filtration) to remove live organisms and propagules associated with macro-organisms (Scianni and Georgiades, 2019). Targeted particle size thresholds range between 2 μm (Morrisey et al., 2013), 5 μm (California Water Boards, 2013), 10 μm (Lewis, 2020), 12.5 μm (Morrisey et al., 2015; Growcott et al., 2019), 50 μm (Department of Agriculture [DOA] et al., 2015), and 60 μm (Morrisey et al., 2013). Assessments of filtration technologies associated with ballast water management systems (BWMS) that use similar designs and functions as those incorporated in RICC systems have shown that they are far from 100% effective at removing live organisms above the stated target particle size, or even the specific physical mesh or sieve size employed (e.g., Gregg et al., 2009; Briski et al., 2014; Cangelosi et al., 2014). Although filtration and settlement can be effective in removing the proportion of pathogens contained within infected material, physical separation of particles, even down to 2–5 um, is unlikely to completely remove many known pathogens, including smaller protists, bacteria, and viruses (Tables 1–3), or dissolved biocides (Terraphase Engineering Inc, 2012; Tamburri et al., 2020), from the effluent. Additional effluent treatment options (i.e., a disinfection step) should therefore be considered where the prevention of pathogen translocation associated with vessel biofouling is a concern.
Additional effluent treatment options for reactive in-water cleaning
For high risk international vessels or vessels from regions with different biosecurity conditions, additional measures to the physical separation of captured debris alone include, but are not limited to, treatment using biocides, UV radiation, or heat to render propagules non-viable (Table 5), or direct disposal of the liquid effluent into municipal sewerage where permitted (Morrisey and Woods, 2015). Pre-filtration to reduce the particulate and organic material present is still required to improve the treatment efficacy of UV, ozone, or oxidants (Chahal et al., 2016; Hess-Erga et al., 2019). Depending on the treatment purpose, pre-filtration recommendations vary between 7 μm (Fraser et al., 2006), 20 μm (Sassi et al., 2005), and 50 μm (Department of Agriculture, Fisheries and Forestry [DAFF], 2008). High flow velocities, however, such as those associated with fouling removal by RICC systems, may decrease filtration efficacy by reducing the contact time between pathogens and particles, and increasing hydraulic shear which can disrupt pathogen-to-particle binding (Chahal et al., 2016). While this may lead to increase in the exposure to subsequent treatments resulting improved efficacy, it also results increased load on this stage of the treatment process.
The concept of appropriate effluent treatment from RICC systems is challenging because:
a) The macrofouling species and pathogens present on submerged surfaces of any given vessel will be largely unknown, therefore any treatments employed should have a wide range of efficacy across a number of pathogen types (e.g., viruses, bacteria, and protists).
b) The efficacy of some treatments (e.g., biocides and UV) is dictated by the amount of organic and particulate matter present and the size of some pathogens (i.e., viruses) is far smaller than any filter size that can be practically achieved. The main purpose of physical separation will, therefore, be to reduce the amount of particulate and organic matter present to assist the efficacy of any subsequent treatment(s).
c) The efficacy of some subsequent treatments (e.g., biocides and UV) will be difficult to ascertain without some form of indicator. That is, approaches for disinfecting effluent prior to discharge should demonstrate their efficacy in removing viral, bacterial, and protistan pathogens (or surrogates for pathogens).
d) Biocidal treatments may be subject to local water quality regulations and may require neutralization prior to effluent discharge.
Effluent treatment for the in-water removal of high-risk biofouling can be guided by lessons learned from municipal sewage treatment (Henze et al., 2008; Chahal et al., 2016), the influent and effluent treatment for land-based aquaculture (Fraser et al., 2006; Department of Agriculture, Fisheries and Forestry [DAFF], 2008; Meyers, 2010; Baulch et al., 2013; Whittington et al., 2020), and the development of BWMS (Balaji et al., 2014; First and Drake, 2014; Batista et al., 2017; Hess-Erga et al., 2019).
While the fundamental goal for management of both the biofouling and ballast water pathways is to minimize the risk of non-indigenous species introductions from vessels, discharge thresholds and management approaches for ballast water regulations (e.g., International Maritime Organization [IMO], 2004) are not directly applicable to RICC of vessel biofouling because:
a) Ballast water brought onboard ships has fewer suspended solids and smaller particle sizes than wastewater produced and treated by RICC.
b) The planktonic organisms treated by BWMS are dissimilar in many ways to micro- and macro-organisms observed within biofouling assemblages.
c) The IMO ballast water regulations do not consider organisms less than 10 μm in size, apart from a few target taxa (E. coli, Enterococci, and toxigenic V. cholerae).
d) RICC systems use large volumes of ambient water in the cleaning process, and RICC effluent therefore requires treatment of biofouling organisms removed from ship surfaces and substantial biomass of local planktonic organisms.
e) Ballast water effluent treatment methods are unlikely to address the environmental risk of releasing antifouling biocides associated with vessel coatings.
In-water cleaning systems do not have the same physical space and power limitations as shipboard BWMS, which could facilitate broader treatment options, such as those used in land-based water treatment, including sewage treatment facilities and aquaculture establishments. While all RICC systems incorporate some form(s) of physical separation (e.g., settling tank, filter, hydrocyclone, and flocculation), decisions about the incorporation of subsequent treatment to either remove dissolved antifouling biocides (e.g., selective metal-binding or adsorption media) or kill pathogens should carefully consider a number of factors including, but not limited to: the marine values requiring protection, risk reduction outcomes, treatment feasibility and practicality, and cost (see Inglis et al., 2012). The following are examples of possible subsequent treatment and disinfection approaches, but not an exhaustive list.
Chlorine
A common disinfectant for wastewater, municipal water, and ballast water (see Ghernaout, 2017), “chlorine”—used here to represent the suite of reactive halogens derived from chlorine—may be suited to disinfect effluent from in-water cleaning operations (Table 5). Chlorine can be injected from an external source, or it may be produced on-site, through electrolytic chlorine generation. Regardless of the chlorine compound, and irrespective of whether it is introduced as a solid, liquid, or a gas, a series of cascading reactions occur based upon the water characteristics following the injection of the compound into the effluent. For example, an electrolytic chlorine generator introduces chlorine as a gas, which upon dissolution in water, reacts to form hypochlorous acid (HOCl). In seawater, HOCl is unstable and quickly dissociates to form hypochlorite (OCl−) and then hypobromous acid, which is also an effective germicide and more stable than HOCl at the pH of seawater (Wong and Davidson, 1977; Abarnou and Miossec, 1992). When ammonia is present in seawater, the oxidants produced through electrolysis form mono-, di-, or tri-chloramines. While these are not considered the primary biocidal agent, some organisms (including invertebrates such as copepods and crab larvae) are sensitive to chloramines (Capuzzo, 1979).
Some BWMS use chlorine to treat large volumes of natural water (Joint Group of Experts on the Scientific Aspects of Marine Environmental Protection [GESAMP], 2019). Generally, ballast water is treated upon uptake, i.e., entry into the ship prior to transit. Treated water is typically held in ballast tanks for at least 1 day, and residual oxidants are neutralized prior to discharge into the environment. In-water cleaning operations require rapid treatment of large volumes, but—relative to ships’ ballasting operations—reservoir volumes are small and hold times are minimal. Adapting a BWMS-like approach for in-water cleaning operations would require large tanks and other infrastructure, which may not be practicable. For active substances, treatment would have to occur following the final filtration, as to minimize organic particulates that consume oxidants. In an in-water cleaning operation, treated water would almost immediately be neutralized thus limiting system efficacy, as short treatment times limit the dispersion of chlorine and the reactivity of the primary oxidizing species. Biocidal concentrations required for high flow, small reservoir systems will exceed those for treating ballast water, and the effective doses would vary based upon a variety of factors including temperature, pH, light, salinity, presence of organic matter (Chahal et al., 2016; Batista et al., 2017; Hess-Erga et al., 2019), and contact time. These factors emphasize the importance of measuring the residual dose of chlorine in the system and assessing efficacy using validated methods.
Chlorine may be effective for effluent treatment if on-site or nearby reservoirs are used to hold treated water, allowing time for the reaction and dissipation of chlorine prior to neutralization. Other strategies to improve the biocidal efficacy of chlorine will allow for shorter residence times in the treatment system. For example, combinations of chlorine and other reagents (e.g., CO2) have shown improved efficacy (Growcott et al., 2017; Hess-Erga et al., 2019). Likewise, mixed-oxidant systems have demonstrated improved biocidal efficacy relative to chlorine alone (Venczel et al., 1997). Finally, an approach for the in-water treatment of hull surfaces has been envisioned: this suggestion would use chlorine produced directly on the hull surfaces by electrolytic chlorination as an antifouling treatment (Iliopoulos et al., 2014). For this approach, ships’ existing cathodic protection systems would be reengineered so that chlorine—naturally produced at the anodes—is dispersed to prevent fouling on the hull and other wetted surfaces. This approach would guard against both macrofouling organisms and their pathogens. However, “in-water chlorination” would potentially introduce high concentrations of chlorine (and disinfection byproducts) into natural water systems which would likely violate the local water quality standards of many jurisdictions.
While chlorination appears to be an obvious candidate for secondary treatment of in-water cleaning effluent, its efficacy remains unresolved (Cahill et al., 2019a), the effluent would need to be neutralized prior to discharge to satisfy local water quality standards, and it is not yet incorporated in any commercially available RICC system.
Ultraviolet (UV) radiation
Ultraviolet radiation is used to disinfect drinking, waste, and ballast water (see Chen et al., 2006). By contrast to chlorine, UV radiation is readily adaptable for an in-line system for effluent treatment: treatment occurs during the short period that suspended organism transits through a chamber. Reactive chemicals are not required, nor are neutralizing agents. Effluent water could be discharged immediately following treatment, given that the dose is sufficient. To achieve the prescribed ballast water discharge standards, Oemcke et al. (2004) and Kim et al. (2019) recommended doses of 60–70 mJ/cm2 to treat most bacteria and viruses (Table 5). Oemcke et al. (2004) further recommended that a dose of 120 mJ/cm2 would remove most micro-organisms except for resistant cysts and viruses. Much higher doses are required to treat the cysts of Cryptosporidium spp. (> 200 mJ/cm2) and diatoms such as Gymnodinium catenatum (> 1,600 mJ/cm2) (Gregg et al., 2009). For many micro-organisms, the effect of UV irradiation may not be immediate, raising the issue of organism viability (i.e., treatment efficacy). This issue has introduced a variety of complications when determining the efficacy of BWMS (First and Drake, 2014; Batista et al., 2017; Peperzak et al., 2020). For example, damage to organisms, in certain cases, may be repairable (e.g., Zimmer and Slawson, 2002). Similar to chlorine, UV radiation efficacy varies based upon the water characteristics, particularly the concentration of particulate and dissolved material (primarily organic matter), which reduce the UV transmissivity (%UVT), but may also scavenge reactive oxidation species (Ou et al., 2011). At least one commercially available RICC system includes the option of secondary UV treatment of captured debris (Lewis, 2013).
Ozone
Ozone is an established water treatment (see Langlais et al., 1991) and is effective against a range of micro-organisms (Gregg et al., 2009; Chahal et al., 2016). Similar to chlorine, ozone is an oxidizing compound that may be generated on-site. For ballast water treatment > 5 mg/L, total residual oxidants for 10 h appear to be a broad-spectrum treatment for free-living bacteria, dinoflagellates, and diatoms; however, 8–14 mg/L for 24 h was required to effectively treat the spore-forming bacteria Bacillus subtilis (see Gregg et al., 2009; Table 5). Ozone—when used as a disinfectant for effluent from a land-based RICC treatment system—has some of the same limitations and caveats as chlorine. Treatment efficacy is a product of both dose concentration and exposure time, and for a flow-through system, exposure time will be limited. Likewise, residual oxidants will require neutralization. Ozone is not yet incorporated into RICC systems.
Heat
Some in-water cleaning technologies use heat as an approach for removing fouling, particularly the algal and biofilm fouling on easily accessible areas of ships’ hulls (Morrisey and Woods, 2015). In this case, heat treatment would be effective against free-living pathogens. Heat may also be used in shore-side treatment systems to kill organisms remaining prior to discharge. For treatment of macrofouled vessel internal seawater systems, Cahill et al. (2019b) and Growcott et al. (2019) recommended exposure to 60°C for 60 min. Such a treatment would appear to be effective against all but the hardiest pathogens, e.g., birnaviruses such as infectious pancreatic necrosis virus (IPNV; Fraser et al., 2006). Aquatic birnaviruses have been found in oysters and mussels located near salmon farms (Mortensen, 1993; Rivas et al., 1993). IPNV has had substantial impacts on global salmonid aquaculture (Munro and Midtlyng, 2011) and has been experimentally transmitted from Mytilus edulis to Salmo salar by cohabitation (Molloy et al., 2013). When considering the range of pathogens of biosecurity relevance to Australia, Diggles (2020) concluded that heating (80°C in water, > 5 min) would meet the acceptable level of protection (i.e., an annual probability of establishment between 1 in 20 and 1 in 100 years) for all identified risk pathogens despite uncertainty from data deficiency (Table 5).
For BWMS, chlorine and UV radiation are common disinfectants, but heat is also used in some cases. For one particular BWMS, the heat source is primarily waste heat from the ship engines and cargo pumps. Treatment occurs in a section of heated pipe, designed so that flowing water meets minimal hold time and temperature limits for disinfection. Note that this system, while shown in Type Approval testing to meet limits on regulated groups of organisms (e.g., Coast Guard Maritime Commons, 2020), was not evaluated for its efficacy in treating pathogenic bacteria, protists < 10 μm, and viruses. While heat treatment is not sensitive to most water characteristics (such as dissolved organic material, salinity, etc.), its efficiency does depend upon the temperature of the input water, i.e., more energy is required to meet the minimum effective temperature in cold and temperate waters relative to tropical waters. On ships, heat-based BWMS may be coupled with heat generating systems, but on a shore-side operation, it is likely that a dedicated, intentional heat source is necessary. The feasibility for using heat to treat the effluent from in-water cleaning operations, therefore, rests on the characteristics of a specific location, including water temperatures, access to fuel or energy for boilers, and opportunities for capturing waste heat. Heat is not yet incorporated into RICC systems.
Conclusion
The long history of devastating impacts to marine values caused by cross-boundary translocation of marine pathogens has resulted in improved practices from regulatory and non-regulatory controls that apply to established pathways, such as fisheries and aquaculture. Available evidence indicates that vessel biofouling is also a viable and important pathway for translocating marine pathogens which presents a risk to marine values. This largely unmanaged pathway, therefore, represents a considerable gap in the biosecurity measures of jurisdictions committed to the prevention and control of aquatic disease. Preventive measures, such as those used in New Zealand and Californian biofouling regulations, lower the likelihood of pathogen translocation by reducing diversity, population size, and total mass of susceptible hosts and carrier species on vessels. Reactive measures, such as in-water removal of macrofouling, may, however, exacerbate the problem and will likely need modification to manage the risk associated with pathogens. While lessons learned from ballast water management, sewage treatment, and aquaculture industries should be considered when developing cleaning and effluent treatment criteria for reactive approaches, preventing or greatly reducing the translocation of pathogens using proactive measures for vessel biofouling is likely to be more effective. Both proactive and reactive solutions, however, have their own unique challenges. The balance between marine protection and risk reduction versus treatment feasibility and cost requires careful consideration.
Data Availability Statement
The original contributions presented in the study are included in the article. Further inquiries can be directed to the corresponding author/s.
Author Contributions
EG and DK conceived the idea for the manuscript which was drafted by EG. DK, CS, ID, MT, MF, GR, KE, and MD revised the manuscript draft and contributed sections based on their areas of expertise. All authors contributed to manuscript revision and read and approved the submitted version.
Funding
Preparation of this article was funded by the Risk Assessment Group, Ministry for Primary Industries, New Zealand. The New Zealand Ministry for Business Innovation and Employment’s Endeavour Fund: Aquaculture Health Strategies to Maximise Productivity and Security (CAWX1707) funded ID’s contribution. The U.S. Department of Transportation’s Maritime Administration supported MT’s and MF’s contributions.
Conflict of Interest
The authors declare that the research was conducted in the absence of any commercial or financial relationships that could be construed as a potential conflict of interest.
Acknowledgments
Draft versions of this manuscript were reviewed by Oliver Quinn, Michael Ormsby, Enrico Perotti (MPI), and Nicole Dobroski (CSLC). Jonathan Thompson (CSLC) provided useful discussions and assistance regarding ballast water management. The authors thank the two reviewers for their constructive feedback which greatly improved the manuscript.
References
Abarnou, A., and Miossec, L. (1992). Chlorinated waters discharged to the marine environment: Chemistry and environmental impact - An overview. Sci. Total Environ. 126, 173–197. doi: 10.1016/0048-9697(92)90490-j
Abollo, E., Ramilo, A., Casas, S. M., Comesaña, P., Cao, A., Carballal, M. J., et al. (2008). First detection of the protozoan parasite Bonamia exitiosa (Haplosporidia) infecting flat oyster Ostrea edulis grown in European waters. Aquaculture 274, 201–207. doi: 10.1016/j.aquaculture.2007.11.037
Alagarswami, K. (1977). “Larval Transport and Settlement of Pearl Oysters (genus Pinctada) in the Gulf of Mannar,” in Proceedings of the Symposium on Warm Water Zooplankton, (Goa: National Institute of Oceanography).
Aldred, N., and Clare, A. S. (2008). The adhesive strategies of cyprids and development of barnacle-resistant marine coatings. Biofouling 24, 351–363. doi: 10.1080/08927010802256117
Alliance for Coastal Technologies Maritime Environmental Resource Center [ACT/MERC] (2019). Evaluation of Subsea Global Solutions in-Water Cleaning and Capture Technology for Ships. Baltimore, MD: ACT/MERC, ACT/MERC IWCC Evaluation Report ER01-19. Available online at: http://www.maritime-enviro.org/Downloads/Reports/Other_Publications/ACT_MERC_SGS_IWCC_Evaluation_Report.pdf (accessed April, 2021).
Amara, I., Miled, W., Slama, R. B., and Ladhari, N. (2018). Antifouling processes and toxicity effects of antifouling paints on marine environment. Environ. Toxicol. Pharm. 57, 115–130. doi: 10.1016/j.etap.2017.12.001
Angell, C. L. (1986). he Biology and Culture of Tropical Oysters. ICLARM Studies and Reviews. Vol. 13. International Center for Living Aquatic Resources Management. Philippines: Manila.
Apte, S., Holland, B. S., Godwin, L. S., and Gardner, J. P. A. (2000). Jumping ship: A stepping stone event mediating transfer of nonindigenous species via a potentially unsuitable environment. Biol. Invas. 2, 75–79.
Arzul, I., Gagnaire, B., Bond, C., Chollet, B., Morga, B., Ferrand, S., et al. (2009). Effects of temperature and salinity on the survival of Bonamia ostreae, a parasite infecting flat oysters Ostrea edulis. Dis. Aquat. Organ. 85, 67–75. doi: 10.3354/dao02047
Arzul, I., Moissec, L., Blanchet, E., Garcia, C., Francois, C., and Joly, J.-P. (2006). “Bonamia ostreae and Ostrea edulis: a Stable Host–parasite System in France?,” in Proceedings of the 11th International Symposium on Veterinary Epidemiology and Economics. Cairns.
Balaji, R., Yaakob, O., and Koh, K. K. (2014). A review of developments in ballast water management. Environ. Rev. 22, 298–310. doi: 10.1139/er-2013-0073
Bailey, S. A., Brown, L., Campbell, M. L., Carlton, J. T., Castro, N., Chainho, P., et al. (2020). Trends in the detection of aquatic non-indigenous species across global marine, estuarine and freshwater ecosystems: A 50−year perspective. Diver. Distribut. 26, 1780–1797. doi: 10.1111/ddi.13167
Barbieri, M., Maltagliati, F., Di Giuseppe, G., Cossu, P., Lardicci, C., and Castelli, A. (2011). New records of the pygmy mussel Xenostrobus securis (Bivalvia: Mytilidae) in brackish-water biotopes of the western Mediterranean provide evidence of its invasive potential. Mar. Biodiv. Rec. 2011:4.
Barkai, A., and Branch, G. M. (1989). Growth and mortality of the mussels Choromytilus meridionalis (Krauss) and Aulacomya ater (Molina) as indicators of biotic conditions. J. Molluscan Stud. 55, 329–342. doi: 10.1093/mollus/55.3.329
Bateman, K. S., Tew, I., French, C., Hicks, R. J., Martin, P., Munro, J., et al. (2012). Susceptibility to infection and pathogenicity of White Spot Disease (WSD) in non-model crustacean host taxa from temperate regions. J. Invert. Pathol. 110, 340–351. doi: 10.1016/j.jip.2012.03.022
Batista, W. R., Fernandes, F. C., Lopes, C. C., Lopes, R. S., Miller, W., and Ruiz, G. (2017). Which ballast water management system will you put aboard? Remnant anxieties: A mini-review. Environments 4:54. doi: 10.3390/environments4030054
Baulch, T., Ellard, K., and Bradshaw, M. (2013). Tasmanian abalone biosecurity project: Implementation phase 1: Biosecurity strategies for abalone processors. J. Shellfish Res. 32, 33–35. doi: 10.2983/035.032.0107
Bell, A., Philipps, S., Denny, C., Georgiades, E., and Kluza, D. (2011). Risk Analysis: Vessel Biofouling. Ministry of Agriculture and Forestry Biosecurity. New Zealand: Wellington.
Bingham, P., Brangenberg, N., Williams, R., and van Andel, M. (2013). Investigation into the first diagnosis of ostreid herpesvirus type 1 in Pacific oysters. Surveillance 40, 20–24.
Bishop, M. J., Carnegie, R. B., Stokes, N. A., Peterson, C. H., and Burreson, E. M. (2006). Complications of a non-native oyster introduction: facilitation of a local parasite. Mar. Ecol. Prog. Series 325, 145–152. doi: 10.3354/meps325145
Bobo, M. Y., Richardson, D. L., Coen, L. D., and Burrell, V. G. (1997). A Report on the Protozoan Pathogens Perkinsus marinus (Dermo) and Haplosporidium nelsoni (MSX) in South Carolina Shellfish Populations. South Carolina Marine Resources Division Technical Report No. 86. Columbia: South Carolina State Library.
Bookelaar, B. E., Reilly, A. O., Lynch, S. A., and Culloty, S. C. (2018). Role of the intertidal predatory shore crab Carcinus maenas in transmission dynamics of ostreid herpesvirus-1 microvariant. Dis. Aquat. Organ. 130, 221–233. doi: 10.3354/dao03264
Bower, S. M. (2019). Synopsis of Infectious Diseases and Parasites of Commercially Exploited Shellfish: Marteilia refringens/maurini of mussels. Available online at: https://www.dfo-mpo.gc.ca/science/aah-saa/diseases-maladies/mrmaurmu-eng.html (accessed September 2020)
Bower, S. M. (2017). Synopsis of Infectious Diseases and Parasites of Commercially Exploited Shellfish: Table of Contents. Available online at: https://www.dfo-mpo.gc.ca/science/aah-saa/diseases-maladies/toc-eng.html#mol (accessed September 2020)
Bower, S. M. (2015). Synopsis of Infectious Diseases and Parasites of Commercially Exploited Shellfish: Bonamia (=Mikrocytos) roughleyi (Australian winter disease) of oysters. Available online at: https://www.dfo-mpo.gc.ca/science/aah-saa/diseases-maladies/mikrouoy-eng.html (accessed September 2020)
Bower, S. M. (2014a). Synopsis of Infectious Diseases and Parasites of Commercially Exploited Shellfish: Haplosporidium costale (SSO) of Oysters. Available online at: https://www.dfo-mpo.gc.ca/science/aah-saa/diseases-maladies/hcoy-eng.html (accessed September 2020)
Bower, S. M. (2014b). Synopsis of Infectious Diseases and Parasites of Commercially Exploited Shellfish: Haplosporidium nelsoni (MSX) of oysters. Available online at: https://dfo-mpo.gc.ca/science/aah-saa/diseases-maladies/hapneloy-eng.html (accessed September 2020).
Bower, S. M. (2014c). Synopsis of Infectious Diseases and Parasites of Commercially Exploited Shellfish: Haplosporidium sp. of rock oysters. Available online at: https://www.dfo-mpo.gc.ca/science/aah-saa/diseases-maladies/haprockoy-eng.html (accessed September 2020)
Bower, S. M. (2013a). Synopsis of Infectious Diseases and Parasites of Commercially Exploited Shellfish: Hematodinium sp. (Bitter Crab Disease). Available online at: https://www.dfo-mpo.gc.ca/science/aah-saa/diseases-maladies/hematcb-eng.html (accessed September 2020)
Bower, S. M. (2013b). Synopsis of Infectious Diseases and Parasites of Commercially Exploited Shellfish: Hematodinium perezi and Hematodinium sp. of Atlantic Crabs. Available online at: https://www.dfo-mpo.gc.ca/science/aah-saa/diseases-maladies/hphacb-eng.html (accessed September 2020)
Bower, S. M., and Kleeman, S. N. (2011). Synopsis of Infectious Diseases and Parasites of Commercially Exploited Shellfish: Marteilia sydneyi of oysters. Available online at: https://www.dfo-mpo.gc.ca/science/aah-saa/diseases-maladies/marsydoy-eng.html (accessed September 2020)
Bower, S. M. (2010). Synopsis of Infectious Diseases and Parasites of Commercially Exploited Shellfish: Juvenile disease of Eastern oysters. Available online at: https://dfo-mpo.gc.ca/science/aah-saa/diseases-maladies/jdeoy-eng.html (accessed September 2020).
Bower, S. M. (2009). Synopsis of Infectious Diseases and Parasites of Commercially Exploited Shellfish: Vibrio spp. (larval and juvenile vibriosis) of oysters. Available online at: https://www.dfo-mpo.gc.ca/science/aah-saa/diseases-maladies/vibrioy-eng.html (accessed September 2020)
Bower, S. M. (2006). Synopsis of Infectious Diseases and Parasites of Commercially Exploited Shellfish: Nocardiosis of Oysters. Available online at: https://dfo-mpo.gc.ca/science/aah-saa/diseases-maladies/nocardoy-eng.html (accessed September 2020).
Bower, S. M. (2001a). Synopsis of Infectious Diseases and Parasites of Commercially Exploited Shellfish: Gill disease of Portuguese oysters. Available online at: https://dfo-mpo.gc.ca/science/aah-saa/diseases-maladies/gilldpoy-eng.html (accessed September 2020).
Bower, S. M. (2001b). Synopsis of Infectious Diseases and Parasites of Commercially Exploited Shellfish: Hinge Ligament Disease of Juvenile Oysters. Available online at: https://www.dfo-mpo.gc.ca/science/aah-saa/diseases-maladies/hldjoy-eng.html (accessed September 2020)
Bower, S. M. (2001c). Synopsis of Infectious Diseases and Parasites of Commercially Exploited Shellfish: Kidney coccidia of mussels. Available online at: https://www.dfo-mpo.gc.ca/science/aah-saa/diseases-maladies/kidcocmu-eng.html (accessed September 2020)
Bower, S. M. (2001d). Synopsis of Infectious Diseases and Parasites of Commercially Exploited Shellfish: Oyster velar virus disease (OVVD). Available online at: https://dfo-mpo.gc.ca/science/aah-saa/diseases-maladies/ovvdoy-eng.html (accessed September 2020).
Bower, S. M. (2001e). Synopsis of Infectious Diseases and Parasites of Commercially Exploited Shellfish: Virus-like disease of mussels. Available online at: https://www.dfo-mpo.gc.ca/science/aah-saa/diseases-maladies/virldmu-eng.html (accessed September 2020)
Bower, S. M., Korrûbel, J. L., and Webb, S. C. (2002). Synopsis of Infectious Diseases and Parasites of Commercially Exploited Shellfish: Phototrophic endolith invasion of mussel shells. Available online at: https://www.dfo-mpo.gc.ca/science/aah-saa/diseases-maladies/bgasimu-eng.html (accessed September 2020)
Bower, S. M., McGladdery, S. E., and Price, I. M. (1994). Synopsis of Infectious Diseases and Parasites of Commercially Exploited Shellfish: Haemocytic infection virus disease of oysters. Available online at: https://dfo-mpo.gc.ca/science/aah-saa/diseases-maladies/hivoy-eng.html (accessed September 2020).
Briski, E., Linley, R. D., Adams, J., and Bailey, S. A. (2014). Evaluating efficacy of a ballast water filtration system for reducing spread of aquatic species in freshwater ecosystems. Manag. Invas. 5, 245–253. doi: 10.3391/mbi.2014.5.3.08
Brock, R., Bailey-Brock, J. H., and Goody, J. (1999). A case study of efficacy of freshwater immersion in controlling introduction of alien marine fouling communities: the USS Missouri. Pacific Sci. 53, 223–231.
Buss, J., Wiltshire, K. H., Harris, J. O., and Deveney, M. R. (2020). Decontamination of Bonamia exitiosa. Aquaculture 2020:735210. doi: 10.1016/j.aquaculture.2020.735210
Cahill, P., Hickey, C., Lewis, P., Tait, L., and Floerl, O. (2019a). Treatment Agents for Biofouling in Internal Pipework of Recreational Vessels. Available online at: https://www.mpi.govt.nz/dmsdocument/33606-treatment-agents-forbiofouling-in-internal-pipework-of-recreational-vessels-a-review-ofpipework-configurations-biofouling-risk-and-operational-considerations (accessed March 2019).
Cahill, P., Tait, L., Floerl, O., Bates, T., Growcott, A., and Georgiades, E. (2019b). Design and assessment of a thermal treatment system for fouled internal pipework of recreational vessels. Mar. Pollut. Bull. 139, 65–73. doi: 10.1016/j.marpolbul.2018.12.032
California Code of Regulations (2017). Biofouling Management to Minimize the Transfer of Nonindigenous Species from Vessels Arriving at California Ports. California, CA: California Code of Regulations.
California Water Boards. (2013). In-water Vessel Hull Cleaning. Best Management Practice Fact Sheet – July 2013. Available online at: http://www.waterboards.ca.gov/sanfranciscobay/publications_forms/documents/In-water_vessel_hull_cleaning_fact_sheet.pdf (accessed September 2020)
Calvo, G. W., Luckenbach, M., and Burreson, E. M. (1999). A Comparative Field Study of Crassostrea gigas and Crassostrea virginica in Relation to Salinity in Virginia. Special Reports in Applied Marine Science and Ocean Engineering (SRAMSOE) No. 349. Williamsburg: Virginia Institute of Marine Science, William and Mary.
Cangelosi, A., Aliff, M., Allinger, L., Balcer, M., Beesley, K., Desai, M., et al. (2014). Results of Shipboard Approval Tests of Ballast Water Treatment Systems in Freshwater (No. CG-D-05-15). New London: Coast Guard Research and Development Center.
Capuzzo, J. M. (1979). Effect of temperature on the toxicity of chlorinated cooling water to marine animals: A preliminary review. Mar. Pollut. Bull. 10, 45–47. doi: 10.1016/0025-326x(79)90267-4
Carlton, J. T. (1999). Molluscan invasions in marine and estuarine communities. Malacologia 41, 439–454.
Casas, S. M., Villalba, A., and Reece, K. S. (2002). Study of perkinsosis in the carpet shell clam Tapes decussatus in Galicia (NW Spain). I. Identification of the aetiological agent and in vitro modulation of zoosporulation by temperature and salinity. Dis. Aquat. Organ. 50, 51–65. doi: 10.3354/dao050051
Centre for Environment, Fisheries and Aquaculture Science [Cefas]. (2009). Shellfish Biosecurity Measures Plan. Guidance and Templates for Shellfish Farmers and Traders. Weymouth: Fish Health Inspectorate, Centre for Environment, Fisheries and Aquaculture Science.
Chahal, C., Van Den Akker, B., Young, F., Franco, C., Blackbeard, J., and Monis, P. (2016). Pathogen and particle associations in wastewater: Significance and implications for treatment and disinfection processes. Adv. Appl. Microb. 97, 63–119.
Chen, J. P., Yang, L., Wang, L. K., and Zhang, B. (2006). “Ultraviolet Radiation for Disinfection,” in Advanced Physicochemical Treatment Processes. Handbook of Environmental Engineering, Vol. 4, eds L. K. Wang, Y. T. Hung, and N. K. Shammas (Totowa, NY: Humana Press).
Choi, K. S., and Park, K. I. (2010). “Review on the Protozoan Parasite Perkinsus olseni (Lester and Davis 1981) Infection in Asian Waters,” in Coastal Environmental and Ecosystem Issues of the East China Sea, eds A. Ishimatsu and H.-J. Lie (Japan: Hiroshima University Press), 269–281.
Coast Guard Maritime Commons (2020). Marine Safety Center issues Ballast Water Management System Type Approval Certificate to BAWAT A/S. Maritime Commons web posting, 3/4/2020. Available online at: https://mariners.coastguard.blog/2020/03/04/marine-safety-center-issues-ballast-water-management-system-type-approval-certificate-to-bawat-a-s/ (accessed January 2021).
Cohen, N. J., Slaten, D. D., Marano, N., Tappero, J. W., Wellman, M., Albert, R. J., et al. (2012). Preventing maritime transfer of toxigenic Vibrio cholerae. Emerg. Infect. Dis. 18, 1680–1682.
Costello, K. E., Lynch, S. A., McAllen, R., O’Riordan, R. M., and Culloty, S. C. (2020). The role of invasive tunicates as reservoirs of molluscan pathogens. Biol. Invas. 2020, 1–15.
Coutts, A. D., and Dodgshun, T. J. (2007). The nature and extent of organisms in vessel sea-chests: A protected mechanism for marine bioinvasions. Mar. Pollut. Bull. 54, 875–886. doi: 10.1016/j.marpolbul.2007.03.011
Coutts, A. D., and Taylor, M. D. (2004). A preliminary investigation of biosecurity risks associated with biofouling on merchant vessels in New Zealand. N. Zeal. J. Mar. Freshwater Res. 38, 215–229. doi: 10.1080/00288330.2004.9517232
Coutts, A. D. M., Moore, K. M., and Hewitt, C. L. (2003). Ships’ sea-chests: an overlooked transfer mechanism for non-indigenous marine species? Mar. Pollut. Bull. 46, 1504–1515.
Culloty, S. C., and Mulcahy, M. F. (2007). Bonamia ostreae in the Native Oyster Ostrea edulis. Mar. Environ. Health Series 2007:29.
Dafforn, K. A., Lewis, J. A., and Johnston, E. L. (2011). Antifouling strategies: History and regulation, ecological impacts and mitigation. Mar. Pollut. Bull. 62, 453–465. doi: 10.1016/j.marpolbul.2011.01.012
Davidson, I., Scianni, C., Hewitt, C., Everett, R., Holm, E., Tamburri, M., et al. (2016). Mini-review: Assessing the drivers of ship biofouling management–aligning industry and biosecurity goals. Biofouling 32, 411–428. doi: 10.1080/08927014.2016.1149572
Davidson, I., Ashton, G., Ruiz, G., Scianni, C., Brown, C., Pagenkopp-Lohan, K., et al. (2013). Richness, Extent, Condition, Reproductive Status, and Parasitism of Fouling Communities on Commercial Vessels. California: Report to the California State Lands Commission, Marine Invasive Species Program.
Davidson, I. C., Brown, C. W., Sytsma, M. D., and Ruiz, G. M. (2009). The role of containerships as transfer mechanisms of marine biofouling species. Biofouling 25, 645–655. doi: 10.1080/08927010903046268
Davidson, I. C., McCann, L. D., Sytsma, M. D., and Ruiz, G. M. (2008). Interrupting a multi-species bioinvasion vector: The efficacy of in-water cleaning for removing biofouling on obsolete vessels. Mar. Pollut. Bull. 56, 1538–1544. doi: 10.1016/j.marpolbul.2008.05.024
Davies, C. E., Batista, F. M., Malkin, S. H., Thomas, J. E., Bryan, C. C., Crocombe, P., et al. (2019). Spatial and temporal disease dynamics of the parasite Hematodinium sp. in shore crabs. Carc. Parasit. Vect. 12:472.
Deveney, M., Roberts, S., Moody, N., Crane, M., and Ellard, K. (2017). “Biofouling as a Long Distance Vector for Pathogens,” in Proceedings of the 4th Fisheries Research and Development Corporation Australasian Aquatic Animal Health and Biosecurity Scientific Conference, ed. M. S. J. Crane (Cairns, Australia).
Department of Agriculture, Fisheries and Forestry [DAFF] (2008). Operational Procedures Manual - Decontamination (Version 1.0). In: Australian Aquatic Veterinary Emergency Plan (AQUAVETPLAN). Canberra, ACT: Australian Government Department of Agriculture, Fisheries and Forestry.
Department of Agriculture, Water and the Environment (2020). Aquatic Animal Diseases Significant to Australia: Identification Field Guide, 5th Edition. Canberra: Australian Government Department of Agriculture, Water and the Environment. Available online at: https://www.agriculture.gov.au/sites/default/files/documents/field-guide-5th-edition.pdf (accessed September 2020)
Department of Agriculture [DOA], Department of the Environment [DOE], and New Zealand Ministry for Primary Industries [MPI]. (2015). Antifouling and in-Water Cleaning Guidelines. Canberra: Department of Agriculture.
Diggles, B. K. (2020). Risk Analysis: Biosecurity Risks Related to Recycling of Mollusc Shell Waste for Shellfish Reef Restoration. New Zealand: DigsFish Services Report DF20-03b for Fisheries Research and Development Corporation.
Ding, Z., Yao, Y., Zhang, F., Wan, J., Sun, M., Liu, H., et al. (2015). The first detection of white spot syndrome virus in naturally infected cultured Chinese mitten crabs, Eriocheir sinensis in China. J. Virolog. Methods 220, 49–54. doi: 10.1016/j.jviromet.2015.04.011
Dobretsov, S. (2010). “Marine biofilms,” in Biofouling, eds S. Dürr and J. C. Thomasson (Oxford: Wiley-Blackwell), 123–136.
Drake, J. M., and Lodge, D. M. (2007). Hull fouling is a risk factor for intercontinental species exchange in aquatic ecosystems. Aquat. Invas. 2, 121–131. doi: 10.3391/ai.2007.2.2.7
Drake, L. A., Doblin, M. A., and Dobbs, F. C. (2007). Potential microbial bioinvasions via ships’ ballast water, sediment, and biofilm. Mar. Pollut. Bull. 55, 333–341. doi: 10.1016/j.marpolbul.2006.11.007
Drake, L. A., Meyer, A. E., Forsberg, R. L., Baier, R. E., Doblin, M. A., Heinemann, S., et al. (2005). Potential invasion of micro-organisms and pathogens via ‘interior hull fouling’: Biofilms inside ballast water tanks. Biol. Invas. 7, 969–982. doi: 10.1007/s10530-004-3001-8
Dungan, C. F., Elston, R. A., and Schiewe, M. H. (1989). Evidence for colonization and destruction of hinge ligaments in cultured juvenile Pacific oysters (Crassostrea gigas) by cytophaga-like bacteria. Appl. Env. Microb. 55, 1128–1135. doi: 10.1128/aem.55.5.1128-1135.1989
Dupont, C. L., Grass, G., and Rensing, C. (2011). Copper toxicity and the origin of bacterial resistance—new insights and applications. Metallomics 3, 1109–1118.
European Food Safety Authority [Efsa]. (2015). European Food Safety Authority Panel on Animal Health Welfare. Scientific Opinion on Oyster Mortality. EFSA J. 13:59.
Farnsworth, M., Culloty, S., Carnegie, R., Diggles, B., Deveney, M., and Michael, K. (2020). Report of the Technical Advisory Group 2019 on a Return to Flat Oyster Farming. 23-27 September 2019. Wellington: Produced for Biosecurity New Zealand.
Farrapeira, C. M. R., Ferreira, G. F. D. A., and Tenório, D. D. O. (2010). Intra-regional transportation of a tugboat fouling community between the ports of Recife and Natal, northeast Brazil. Brazilian J. Oceanogr. 58, 1–14.
First, M. R., and Drake, L. A. (2014). Life after treatment: detecting living micro-organisms following exposure to UV light and chlorine dioxide. J. Appl. Phycol. 26, 227–235. doi: 10.1007/s10811-013-0049-9
Fisheries Research and Development Corporation (2011). Final Report, OsHV-1 μ-var International Workshop, Australi: Fisheries Research and Development Corporation.
Flemming, H. C. (2002). Biofouling in water systems–cases, causes and countermeasures. Appl. Microb. Biotechnol. 59, 629–640. doi: 10.1007/s00253-002-1066-9
Floerl, O., Inglis, G. J., Dey, K., and Smith, A. (2009). The importance of transport hubs in stepping-stone invasions. J. Appl. Ecol. 46, 37–45. doi: 10.1111/j.1365-2664.2008.01540.x
Fraser, D. I., Munro, P. D., and Smail, D. A. (2006). Disinfection Guide Version IV. Practical steps to prevent the introduction and minimise the transmission of diseases of fish. Fisheries Research Services Internal Report No. 13/06. In: Code of Good Practice Management Group (2015). The Code of Good Practice for Scottish Finfish Aquaculture. Scotland: Scottish Salmon Producer’s Organisation. Available online at: http://www.thecodeofgoodpractice.co.uk/ (accessed September 2020)
Fuhrmann, M., and Hick, P. M. (2020). Vessel Biofouling and Aquatic Pathogens. Biosecurity new Zealand Technical Paper. New Zealand: Ministry for Primary Industries.
Gadd, J., Depree, C., and Hickey, C. (2011). Relevance to New Zealand of the OECD Emission Scenario Document for Antifouling Paints: Phase 2 Report. Report for the Environmental Protection Authority (EPA). Hamilton: National Institute of Water and Atmospheric Research Ltd.
Georgiades, E. (2015). Risk Assessment: Pathways Associated with Domestic Spread of Bonamia ostreae. Prepared for Animal and Marine Biosecurity Response Team. New Zealand: Ministry for Primary Industries.
Georgiades, E., and Kluza, D. (2017). Evidence-based decision making to underpin the thresholds in New Zealand’s CRMS: biofouling on vessels arriving to New Zealand. J. Mar. Sci. Technol. 51, 76–88. doi: 10.4031/mtsj.51.2.5
Georgiades, E., Kluza, D., Bates, T., Lubarsky, K., Brunton, J., Growcott, A., et al. (2020). Regulating vessel biofouling to support New Zealand’s marine biosecurity system – A blue print for evidence-based decision making. Front. Mar. Sci. 7:390.
Georgiades, E., Growcott, A., and Kluza, D. (2018). Technical Guidance on Biofouling Management for Vessels Arriving to New Zealand. Wellington: Ministry for Primary Industries, 16.
Georgiades, E., Fraser, R., and Jones, B. (2016). Options to Strengthen On-farm Biosecurity Management for Commerical and Non-commercial Aquaculture. Technical Paper No. 2016/47. Wellington: Ministry for Primary Industries.
Ghernaout, D. (2017). Water treatment chlorination: An updated mechanistic insight review. Chem. Res. J. 2, 125–138.
Gias, E., and Johnston, C. (2010). Molecular tools for diagnosis of mollusc pathogens. Surveillance 37, 44–48.
Goedknegt, M. A., Feis, M. E., Wegner, K. M., Luttikhuizen, P. C., Buschbaum, C., Camphuysen, K., et al. (2016). Parasites and marine invasions: Ecological and evolutionary perspectives. J. Sea Res. 113, 11–27.
Goulletquer, P. (2004). Cultured Aquatic Species Information programme. Ostrea edulis. Rome: FAO Fisheries Division [online].
Green, T. J., and Barnes, A. C. (2010). Bacterial diversity of the digestive gland of Sydney rock oysters, Saccostrea glomerata infected with the paramyxean parasite, Marteilia sydneyi. J. Appl. Microb. 109, 613–622.
Gregg, M., Rigby, G., and Hallegraeff, G. M. (2009). Review of two decades of progress in the development of management options for reducing or eradicating phytoplankton, zooplankton and bacteria in ship’s ballast water. Aquat. Invas. 4, 521–565. doi: 10.3391/ai.2009.4.3.14
Groth, S., and Rumrill, S. (2009). History of Olympia oysters (Ostrea lurida Carpenter 1864) in Oregon estuaries, and a description of recovering populations in Coos Bay. J. Shellfish Res. 28, 51–58. doi: 10.2983/035.028.0111
Growcott, A., Kluza, D., and Georgiades, E. (2019). Technical Advice: Evaluation of In-Water Systems to Reactively Treat or Remove Biofouling within Vessel Internal Niche Areas. Technical Paper No. 2019/02. Wellington: Ministry for Primary Industries.
Growcott, A., Kluza, D., and Georgiades, E. (2017). Review: in-water systems to reactively manage biofouling in sea chests and internal pipework. J. Mar. Sci. Technol. 51, 89–104. doi: 10.4031/mtsj.51.2.3
Habe, S., Lai, K. P., Agatsuma, T., Ow-Yang, C. K., and Kawashima, K. (1993). Crab hosts for Paragonimus westermani (Kerbert, 1878) in Malaysia. Japan. J. Trop. Med. Hygiene 21, 137–142. doi: 10.2149/tmh1973.21.137
Hamaguchi, M., Manabe, M., Kajihara, N., Shimabukuro, H., Yamada, Y., and Nishi, E. (2017). DNA barcoding of flat oyster species reveals the presence of Ostrea stentina Payraudeau, 1826 (Bivalvia: Ostreidae) in Japan. Mar. Biodiv. Rec. 10:4.
Hamaguchi, M., Shimabukuro, H., Kawane, M., and Hamaguchi, T. (2013). A new record of the Kumamoto oyster Crassostrea sikamea in the Seto Inland Sea, Japan. Mar. Biodiv. Rec. 2013:6.
Haskin, H. H., and Andrews, J. D. (1988). Uncertainties and speculations about the life cycle of the eastern oyster pathogen Haplosporidium nelsoni (MSX). Am. Fisheries Soc. Special Public. 18, 5–22.
Haskin, H. H., and Ford, S. E. (1982). Haplosporidium nelsoni (MSX) on Delaware Bay seed oyster beds: A host–parasite relationship along a salinity gradient. J. Invertebr. Pathol. 40, 388–405. doi: 10.1016/0022-2011(82)90178-1
Henze, M., van Loosdrecht, M. C. M., Ekama, G. A., and Brdjanovic, D. (eds) (2008). Biological Wastewater Treatment - Principles, Modelling and Design. London: IWA Publishing.
Herborg, L. M., Rushton, S. P., Clare, A. S., and Bentley, M. G. (2003). Spread of the Chinese Mitten Crab (Eriocheir sinensis H. Milne Edwards) in Continental Europe: Analysis of a Historical Data Set. In Migrations and Dispersal of Marine Organisms. Dordrecht: Springer, 21–28.
Hess-Erga, O. K., Moreno-Andrés, J., and Enger, Ø, and Vadstein, O. (2019). Micro-Organisms in ballast water: Disinfection, community dynamics, and implications for management. Sci. Total Environ. 657, 704–716. doi: 10.1016/j.scitotenv.2018.12.004
Hewitt, C. L., and Campbell, M. (2010). The Relative Contribution of Vectors to the Introduction and Translocation of Invasive Marine Species. Commissioned by The Department of Agriculture. Canberra: Fisheries and Forestry (DAFF).
Hick, P., Evans, O., Looi, R., English, C., and Whittington, R. J. (2016). Stability of ostreid herpesvirus-1 (OsHV-1) and assessment of disinfection of seawater and oyster tissues using a bioassay. Aquaculture 450, 412–421. doi: 10.1016/j.aquaculture.2015.08.025
Hill-Spanik, K. M., McDowell, J. R., Stokes, N. A., Reece, K. S., Burreson, E. M., and Carnegie, R. B. (2015). Phylogeographic perspective on the distribution and dispersal of a marine pathogen, the oyster parasite Bonamia exitiosa. Mar. Ecol. Prog. Series 536, 65–76. doi: 10.3354/meps11425
Hine, P. M. (1996). Southern hemisphere mollusc diseases and an overview of associated risk assessment problems. Revue Sci. Tech.-Off. Int. des Èpizooties 15, 563–571. doi: 10.20506/rst.15.2.940
Hine, P. M. (1995). Other mechanisms of marine organism transfer. In: Ballast Water a Marine Cocktail on the Move. R. Soc. N. Zealand Miscell. Series 30, 95–101.
Howard, A. E. (1994). The possibility of long-distance transmission of Bonamia by fouling on boat hulls. Bull. Eur. Assoc. Fish Pathol. 14, 211–212.
Hunsucker, K. Z., Ralston, E., Gardner, H., and Swain, G. (2019). “Specialized grooming as a mechanical method to prevent marine invasive species recruitment and transport on ship hulls,” in Impacts of Invasive Species on Coastal Environments, eds C. Makowski and C. W. Finkl (Berlin: Springer), 247–265. doi: 10.1007/978-3-319-91382-7_7
Iliopoulos, A., Michopoulos, J. G., DeGiorgi, V., and Policastro, S. (2014). “Towards a Computational Multiphysics Framework for Modeling Antibiofouling Processes,” in Proceedings of the International Design Engineering Technical Conferences and Computers and Information in Engineering Conference. New York, NY, Vol. 1A.
Inglis, G. J., Morrisey, D., Woods, C., Sinner, J., and Newton, M. (2013). Managing the Domestic Spread of Harmful Marine Organisms. Part A: Operational Tools for Management. Wellington: Ministry for Primary Industries.
Inglis, G. J., Floerl, O., and Woods, C. (2012). Scenarios of Vessel Biofouling Risk and their Management: An Evaluation of Options. Technical Paper No: 2012/07. Wellington: Ministry of Agriculture and Forestry.
International Maritime Organization [Imo]. (2011). Guidelines for the Control and Management of Ships’ Biofouling to Minimize the Transfer of Invasive Aquatic Species. London: International Maritime Organization.
International Maritime Organization [Imo]. (2004). International Convention for the Control and Management of Ships’ Ballast Water and Sediments. London: International Maritime Organization.
Itoh, N., Komatsu, Y., Maeda, K., Hirase, S., and Yoshinaga, T. (2019). First discovery of Perkinsus beihaiensis in Mediterranean mussels (Mytilus galloprovincialis) in Tokyo Bay, Japan. J. Invertebr. Pathol. 166, 107226. doi: 10.1016/j.jip.2019.107226
Itoh, N., Komiyama, H., Ueki, N., and Orawa, K. (2004). Early developmental stages of a protozoan parasite, Marteilioides chungmuensis (Paramyxea), the causative agent of the ovary enlargement disease in the Pacific oyster, Crassostrea gigas. Internat. J. Parasitol. 34, 1129–1135. doi: 10.1016/j.ijpara.2004.06.001
Johnston, C. J. (2014). Statement of Evidence on Behalf of Fisheries Submitters before the Environmental Protection Authority, Victoria: Environmental Protection Authority.
Johnston, E. L., Dafforn, K. A., Clark, G. F., Rius, M., and Floerl, O. (2017). Anthropogenic activities promoting the establishment and spread of marine non-indigenous species post-arrival. Oceanogr. Mar. Biol. Ann. Rev. 55, 389–420. doi: 10.1201/b21944-6
Joint Group of Experts on the Scientific Aspects of Marine Environmental Protection [GESAMP] (2019). Methodology for the evaluation of ballast water management systems using Active Substances. Reports and Studies GESAMP No. 101.
Keeling, S. E., Brosnahan, C. L., Williams, R., Gias, E., Hannah, M., Bueno, R., et al. (2014). New Zealand juvenile oyster mortality associated with ostreid herpesvirus 1 - An opportunistic longitudinal study. Dis. Aquat. Organ. 109, 231–239. doi: 10.3354/dao02735
Kemp, W. M., Boynton, W. R., Adolf, J. E., Boesch, D. F., Boicourt, W. C., Brush, G., et al. (2005). Eutrophication of Chesapeake Bay: Historical trends and ecological interactions. Mar. Ecol. Prog. Series 303, 1–29. doi: 10.3354/meps303001
Kim, Y., Snow, S. D., Reichel-Deland, V., Maghsoodi, M., Langlois, G. M., Tarabara, V. V., et al. (2019). Current status and recommendations toward a virus standard for ballast water. Manag. Biol. Invasions 10, 267–284. doi: 10.3391/mbi.2019.10.2.04
Kroeck, M. A., and Montes, J. (2005). Occurrence of the haemocyte parasite Bonamia sp. in flat oysters Ostrea puelchana farmed in San Antonio Bay (Argentina). Dis. Aquat. Organ. 63, 231–235. doi: 10.3354/dao063231
Lallias, D., Arzul, I., Heurtebise, S., Ferrand, S., Chollet, B., Robert, M., et al. (2008). Bonamia ostreae-induced mortalities in one-year old European flat oysters Ostrea edulis: Experimental infection by cohabitation challenge. Aquat. Living Res. 21, 423–439. doi: 10.1051/alr:2008053
Lane, H. S., Brosnahan, C. L., and Poulin, R. (2020). Aquatic disease in New Zealand: Synthesis and future directions. N. Zealand J. Mar. Freshwater Res. 2020, 1–42. doi: 10.1080/00288330.2020.1848887
Lane, H. S., and Jones, J. B. (2020). Low internal transcribed spacer rDNA variation in New Zealand Bonamia ostreae: Evidence for a recent arrival. Dis. Aquat. Organ. 139, 121–130.
Lane, H. S., Jones, B., and Poulin, R. (2018). Comparative population genetic study of an important marine parasite from New Zealand flat oysters. Mar. Biol. 165:9.
Lane, H. S., Webb, S. C., and Duncan, J. (2016). Bonamia ostreae in the New Zealand oyster Ostrea chilensis: A new host and geographic record for this haplosporidian parasite. Dis. Aquat. Organ. 118, 55–63. doi: 10.3354/dao02960
Langlais, B., Reckhow, D. A., and Brink, D. R. (1991). Ozone in Water Treatment, Application and Engineering. Boca Raton: CRC Press.
Lewis, J. A. (2020). Chemical Contaminant Risks Associated with In-water Cleaning of Vessels. Department of Agriculture. Canberra: Water and the Environment.
Lewis, J. A. (1986). Fouling Settlement at HMAS Stirling (Cockburn Sound, Western Australia) - A Review (No. MRL-R-995). Australia: Defence Science and Technology Organisation Materials Research Laboratories, Ascot Vale.
Lewis, J. A. (1982). A Guide to the Principal Marine Fouling Organisms, with Particular Reference to Cockburn Sound, WA (No. MRL-R-858). Australia: Defence Science and Technology Organisation Materials Research Laboratories, Ascot Vale.
Lewis, J. A. (2013). In-Water Hull Cleaning and Filtration System: in-Water Cleaning Trials – 26–28 November 2012. Available online at: http://www.fish.wa.gov.au/Documents/occasional_publications/fop114.pdf (accessed July 2013).
Lockwood, J. L., Cassey, P., and Blackburn, T. (2005). The role of propagule pressure in explaining species invasions. Trends Ecol. Evol. 20, 223–228. doi: 10.1016/j.tree.2005.02.004
Longshaw, M., Stone, D. M., Wood, G., Green, M. J., and White, P. (2013). Detection of Bonamia exitiosa (Haplosporidia) in European flat oysters Ostrea edulis cultivated in mainland Britain. Dis. Aquat. Organ. 106, 173–179. doi: 10.3354/dao02643
Lopez-Joven, C., Rolland, J. L., Haffner, P., Caro, A., Roques, C., Carré, C., et al. (2018). Oyster farming, temperature, and plankton influence the dynamics of pathogenic Vibrios in the Thau Lagoon. Front. Microb. 9:2530.
Mann, R. L., Burreson, E. M., and Baker, P. K. (1991). The decline of the Virginia oyster fishery in Chesapeake Bay considerations for introduction of a non-endemic species, Crassostrea gigas (Thunberg, 1793). J. Shell. Res. 10, 379–388.
Martenot, C., Denechere, L., Hubert, P., Metayer, L., Oden, E., Trancart, S., et al. (2015). Virulence of ostreid herpesvirus 1 mu Var in sea water at 16 degrees C and 25 degrees C. Aquaculture 439, 1–6. doi: 10.1016/j.aquaculture.2015.01.012
McCarthy, S. A., and Khambaty, F. M. (1994). International dissemination of epidemic Vibrio cholerae by cargo ship ballast and other nonpotable waters. Appl. Env. Microb. 60, 2597–2601. doi: 10.1128/aem.60.7.2597-2601.1994
McClay, T., Zabin, C., Davidson, I., Young, R., and Elam, D. (2015). Vessel biofouling prevention and management options report. Available online at: https://apps.dtic.mil/dtic/tr/fulltext/u2/a626612.pdf (accessed January 2021).
Meuriot, E., and Grizel, H. (1984). Note sur l’impact conomique des maladies de l’hu tre plate en Bretagne. Rapports Techniques de l Institut Scientifique and Technique des Pêches Maritimes 12, 1-20. France.
Meyers, T. (2010). Regulation Changes, Policies and Guidelines for Alaska Fish and Shellfish Health and Disease Control. Alaska Department of Fish and Game, Regional Information Report 5J10-01, Juneau.
Ministry for Primary Industries New Zealand [Mpi]. (2018). Craft Risk Management Standard: Biofouling on Vessels Arriving to New Zealand. CRMS - BIOFOUL. Wellington: Ministry for Primary Industries.
Molino, P. J., and Wetherbee, R. (2008). The biology of biofouling diatoms and their role in the development of microbial slimes. Biofouling 24, 365–379. doi: 10.1080/08927010802254583
Molloy, S. D., Pietrak, M. R., Bricknell, I., and Bouchard, D. A. (2013). Experimental transmission of infectious pancreatic necrosis virus from the blue mussel, Mytilus edulis, to cohabitating Atlantic salmon (Salmo salar) smolts. Appl. Environ. Microb. 79, 5882–5890. doi: 10.1128/aem.01142-13
Moreau, P., Faury, N., Burgeot, T., and Renault, T. (2015). Pesticides and ostreid herpesvirus 1 infection in the Pacific oyster, Crassostrea gigas. PLoS One 10:e0130628. doi: 10.1371/journal.pone.0130628
Morrisey, D., Inglis, G., Tait, L., Woods, C., Lewis, J., and Georgiades, E. (2015). Procedures for Evaluating In-Water Systems to Remove or Treat Vessel Biofouling. New Zealand Ministry for Primary Industries Technical Paper No. 2015/39. Wellington: Ministry for Primary Industries.
Morrisey, D., and Woods, C. (2015). In-Water Cleaning Technologies: Review of Information. New Zealand Ministry for Primary Industries Technical Paper No. 2015/38. Wellington: Ministry for Primary Industries.
Morrisey, D., Gadd, J., Page, M., Lewis, J., Bell, A., and Georgiades, E. (2013). In-Water Cleaning of Vessels: Biosecurity and Chemical Contamination Risks. New Zealand Ministry for Primary Industries Technical Paper No. 2013/11. Wellington: Ministry for Primary Industries.
Mortensen, S. H. (1993). Passage of infectious pancreatic necrosis virus (IPNV) through invertebrates in an aquatic food chain. Dis. Aquat. Organ. 16, 41–45. doi: 10.3354/dao016041
Moshchenko, A. V., and Zvyagintsev, A. Y. (2001). Composition and structure of macrofouling communities on ocean-going ships of Far East Sea Basin. Ocean Polar Res. 23, 63–75.
Munro, E. S., and Midtlyng, P. J. (2011). “Infectious Pancreatic Necrosis and Associated Aquatic Birnaviruses,” in Fish Diseases and Disorders. Volume 3: Viral, Bacterial and Fungal Infections, Second Edition, eds P. T. K. Woo and D. W. Bruno Wallingford: CABI Publishing. 1–65. doi: 10.1079/9781845935542.0001
National Research Council. (2004). Non-native Oysters in the Chesapeake Bay. Washington, DC: The National Academies Press.
OIE (2020). OIE-Listed diseases, infections and infestations in force in 2020. Available online at: https://www.oie.int/animal-health-in-the-world/oie-listed-diseases-2020/ (accessed September 2020)
OIE (2019). Aquatic Manual. Available online at: https://www.oie.int/en/standard-setting/aquatic-manual/ (accessed September 2020)
Ojaveer, H., Galil, B. S., Carlton, J. T., Alleway, H., Goulletquer, P., Lehtiniemi, M., et al. (2018). Historical baselines in marine bioinvasions: Implications for policy and management. PLoS One 13:e0202383. doi: 10.1371/journal.pone.0202383
Oemcke, D., Parker, N., and Mountfort, D. (2004). Effect of UV irradiation on viability of micro scale and resistant forms of marine organisms: Implications for the treatment of ships’ ballast water. J. Mar. Env. Eng. 7, 153–171.
O’Reilly, A., Laide, C., Maloy, A., Hutton, S., Bookelaar, B. K., Lynch, S. A., et al. (2018). Parasitology 145, 1095–1104.
Ou, H., Gao, N., Deng, Y., Wang, H., and Zhanga, H. (2011). Inactivation and degradation of Microcystis aeruginosa by UV-C irradiation. Chemosphere 85, 1192–1198. doi: 10.1016/j.chemosphere.2011.07.062
Pagenkopp-Lohan, K. M., Ruiz, G. M., and Torchin, M. E. (2020). “Invasions Can Drive Marine Disease Dynamics,” in Marine Disease Ecology, eds D. C. Behringer, K. D. Lafferty, and B. D. Silliman (Oxford: Oxford University Press)115–138. doi: 10.1093/oso/9780198821632.003.0007
Pagenkopp-Lohan, K. M., Hill-Spanik, K. M., Torchin, M. E., Fleischer, R. C., Carnegie, R. B., Reece, K. S., et al. (2018). Phylogeography and connectivity of molluscan parasites: Perkinsus spp. in Panama and beyond. Int. J. Parasitol. 48, 135–144. doi: 10.1016/j.ijpara.2017.08.014
Paul-Pont, I., Evans, O., Dhand, N. K., Rubio, A., Coad, P., and Whittington, R. J. (2014). Descriptive epidemiology of mass mortality due to ostreid herpesvirus-1 (OsHV-1) in commercially farmed Pacific oysters (Crassostrea gigas) in the Hawkesbury River estuary. Australia. Aquaculture 422, 146–159. doi: 10.1016/j.aquaculture.2013.12.009
Peperzak, L., Zetsche, E.-M., Gollasch, S., Artigas, L. F., Bonato, S., Creach, V., et al. (2020). Comparing flow cytometry and microscopy in the quantification of vital aquatic organisms in ballast water. J. Mar. Eng. Tech. 19, 68–77. doi: 10.1080/20464177.2018.1525806
Pepin, J. F., Riou, A., and Renault, T. (2008). Rapid and sensitive detection of ostreid herpesvirus 1 in oyster samples by real-time PCR. J. Virol. Methods 149, 269–276. doi: 10.1016/j.jviromet.2008.01.022
Raynard, R., Wahli, T., Vatsos, I., and Mortensen, S. (eds) (2007). Review of Disease Interactions and Pathogen Exchange between Farmed and Wild Finfish and Shellfish in Europe. Work package 1, deliverable 1.5. Disease Interactions and Pathogen Exchange Between Farmed and Wild Aquatic Animal Populations - a European Network. Issued by Veterinæmedisinsk Oppdragssenter AS. Project number: 1655. Oslo: Veterinrrnedisink Oppdragssenter AS
Revilla-Castellanos, V. J., Guerrero, A., Gomez-Gil, B., Navarro-Barrón, E., and Lizárraga-Partida, M. L. (2015). Pathogenic Vibrio parahaemolyticus isolated from biofouling on commercial vessels and harbor structures. Biofouling 31, 275–282. doi: 10.1080/08927014.2015.1038526
Ricciardi, A., Iacarella, J. C., Aldridge, D. C., Blackburn, T. M., Carlton, J. T., Catford, J. A., et al. (2020). Four priority areas to advance invasion science in the face of rapid environmental change. Env. Rev. 2020:0088. doi: 10.1139/er-2020-0088
Richir, J., Bray, S., McAleese, T., and Watson, G. J. (2021). Three decades of trace element sediment contamination: The mining of governmental databases and the need to address hidden sources for clean and healthy seas. Env. Int. 149:106362. doi: 10.1016/j.envint.2020.106362
Rivas, C., Cepeda, C., Dopazo, C. P., Novoa, B., Noya, M., and Barja, J. L. (1993). Marine environment as reservoir of birnaviruses from poikilothermic animals. Aquaculture 115, 183–194. doi: 10.1016/0044-8486(93)90135-l
Ruiz, G. M., Fofonoff, P. W., Steves, B. P., and Carlton, J. T. (2015). Invasion history and vector dynamics in coastal marine ecosystems: A North American perspective. Aquat. Ecosyst. Health Manag. 18, 299–311. doi: 10.1080/14634988.2015.1027534
Ruiz, G. M., Freestone, A. L., Fofonoff, P. W., and Simkanin, C. (2009). “Habitat Distribution and Heterogeneity in Marine Invasion Dynamics: The Importance of Hard Substrate and Artificial Structure,” in Marine Hard Bottom Communities, ed. M. Wahl (Berlin: Springer-Verlag), 321–332. doi: 10.1007/b76710_23
Ruiz, G. M., Fofonoff, P. W., Carlton, J. T., Wonham, M. J., and Hines, A. H. (2000b). Invasion of coastal marine communities in North America: Apparent patterns, processes, and biases. Ann. Rev. Ecol. Syst. 31, 481–531. doi: 10.1146/annurev.ecolsys.31.1.481
Ruiz, G. M., Rawlings, T. K., Dobbs, F. C., Drake, L. A., Mullady, T., Huq, A., et al. (2000a). Global spread of micro-organisms by ships. Nature 408, 49–50. doi: 10.1038/35040695
Ruiz, G. M., Carlton, J. T., Grosholz, E. D., and Hines, A. H. (1997). Global invasions of marine and estuarine habitats by non-indigenous species: mechanisms, extent, and consequences. Am. Zool. 37, 621–632. doi: 10.1093/icb/37.6.621
Sassi, J., Viitasalo, S., Rytkönen, J., and Leppäkoski, E. (2005). Experiments with Ultraviolet Light, Ultrasound and Ozone Technologies for Onboard Ballast Water Treatment. Espoo 2005. VTT Tiedotteita. Res. 2005:7.
Sauvage, C., Pepin, J. F., Lapegue, S., Boudry, P., and Renault, T. (2009). Ostreid herpes virus 1 infection in families of the Pacific oyster, Crassostrea gigas, during a summer mortality outbreak: differences in viral DNA detection and quantification using real-time PCR. Virus Res. 142, 181–187. doi: 10.1016/j.virusres.2009.02.013
Schultz, M. P., Bendick, J. A., Holm, E. R., and Hertel, W. M. (2011). Economic impact of biofouling on a naval surface ship. Biofouling 27, 87–98. doi: 10.1080/08927014.2010.542809
Schwindt, E., Gappa, J. L., Raffo, M. P., Tatián, M., Bortolus, A., Orensanz, J. M., et al. (2014). Marine fouling invasions in ports of Patagonia (Argentina) with implications for legislation and monitoring programs. Mar. Env. Res. 99, 60–68. doi: 10.1016/j.marenvres.2014.06.006
Scianni, C., Lubarsky, K., Ceballos-Osuna, L., and Bates, T. (in press). Yes, we CANZ: Initial compliance and lessons learned from regulating vessel biofouling management in California and New Zealand. Manag. Biol. Invas.
Scianni, C., and Georgiades, E. (2019). Vessel in-water cleaning or treatment: Identification of environmental risks and science needs for evidence-based decision making. Front. Mar. Sci. 6:467.
Ségarra, A., Pépin, J. F., Arzul, I., Morga, B., Faury, N., and Renault, T. (2010). Detection and description of a particular ostreid herpesvirus 1 genotype associated with massive mortality outbreaks of Pacific oysters, Crassostrea gigas, in France in 2008. Virus Res. 153, 92–99. doi: 10.1016/j.virusres.2010.07.011
Shikuma, N. J., and Hadfield, M. G. (2010). Marine biofilms on submerged surfaces are a reservoir for Escherichia coli and Vibrio cholerae. Biofouling 26, 39–46. doi: 10.1080/08927010903282814
Sim-Smith, C., Faire, S., and Lees, A. (2016). Managing Biosecurity for Business Benefit. Aquaculture Biosecurity Practices Research. Technical Paper No. 2016/14. Wellington: Ministry for Primary Industries.
Smith, M., Inglis, G. J., Wilkens, S., and McDonald, S. (2016). Emergency surveillance for marine pests after the grounding of the container vessel, MV Rena. N. Zealand J. Mar. Freshwater Res. 50, 42–55. doi: 10.1080/00288330.2015.1127828
Stavrakakis, C., Papin, M., Dupuy, B., Riou, K., Penisson, C., and Nourry, M. (2017). Désinfection de l’eau de mer–DESIMER Etude des sous-produits de désinfection. France: Département Ressources Biologiques et Environnement.
Stentiford, G. D., Bateman, K. S., Dubuffet, A., Chambers, E., and Stone, D. M. (2011). Hepatospora eriocheir (Wang and Chen, 2007) gen. et comb. nov. infecting invasive Chinese mitten crabs (Eriocheir sinensis) in Europe. J. Invertebr. Pathol. 108, 156–166. doi: 10.1016/j.jip.2011.07.008
Stephenson, M., and Petrie, B. (2005). Oceanographic influences on the management of MSX disease of American oysters (Crassostrea virginica) in Atlantic Canada. Bull. Aquac. Assoc. Can. 105, 67–78.
Tamburri, M. N., Davidson, I. C., First, M. R., Scianni, C., Newcomer, K., Inglis, G. J., et al. (2020). In-water cleaning and capture to remove ship biofouling: An initial evaluation of efficacy and environmental safety. Front. Mar. Sci. 7:437.
Tamburri, M. N., Luckenbach, M. W., Breitburg, D. L., and Bonniwell, S. M. (2008). Settlement of Crassostrea ariakensis larvae: Effects of substrate, biofilms, sediment and adult chemical cues. J. Shellfish Res. 27, 601–608. doi: 10.2983/0730-8000(2008)27[601:socale]2.0.co;2
Tao, Y., and Wenxia, Y. (2002). Study of offshore fouling in China – now and in the future. Studia Marina Sinica. Available online at: http://en.cnki.com.cn/Article_en/CJFDTotal-HKJK200200011.htm (accessed September 2020)
Terraphase Engineering Inc (2012). In-Water Hull Cleaning Summary Report. Alameda, CA: US-Department of Transportation – Maritime Administration, 48.
Tribou, M., and Swain, G. (2017). The effects of grooming on a copper ablative coating: A six year study. Biofouling 33, 494–504. doi: 10.1080/08927014.2017.1328596
Ulman, A., Ferrario, J., Occhipinti-Ambrogi, A., Arvanitidis, C., Bandi, A., Bertolino, M., et al. (2017). A massive update of non-indigenous species records in Mediterranean marinas. PeerJ 5:e3954. doi: 10.7717/peerj.3954
van Banning, P. (1991). Observations on bonamiasis in the stock of the European flat oyster, Ostrea edulis, in the Netherlands, with special reference to the recent developments in Lake Grevelingen. Aquaculture 93, 205–211. doi: 10.1016/0044-8486(91)90232-v
Venczel, L. V., Arrowood, M., Hurd, M., and Sobsey, M. D. (1997). Inactivation of Cryptosporidium parvum oocysts and Clostridium perfringens spores by a mixed-oxidant disinfectant and by free chlorine. Appl. Env. Microb. 63, 1598–1601. doi: 10.1128/aem.63.4.1598-1601.1997
Vigneron, V., Solliec, G., Montanié, H., and Renault, T. (2004). Detection of ostreid herpesvirus 1 (OsHV-1) DNA in seawater by PCR: Influence of water parameters in bioassays. Dis. Aquat. Organ. 62, 35–44. doi: 10.3354/dao062035
Villalba, A., Reece, K. S., Ordás, M. C., Casas, S. M., and Figueras, A. (2004). Perkinsosis in molluscs: A review. Aquat. Living Resour. 17, 411–432. doi: 10.1051/alr:2004050
Visscher, J. P. (1928). Nature and extent of fouling of ships’ bottoms. Bull. Bureau Fisheries 43, 193–252.
Wang, T., and Li, Q. (2020). Effects of temperature, salinity and body size on the physiological responses of the Iwagaki oyster Crassostrea nippona. Aquac. Res. 51, 728–737. doi: 10.1111/are.14423
Wang, W., Gu, W., Gasparich, G. E., Bi, K., Ou, J., Meng, Q., et al. (2011). Spiroplasma eriocheiris sp. nov., associated with mortality in the Chinese mitten crab, Eriocheir sinensis. Int. J. Syst. Evol. Microb. 61, 703–708. doi: 10.1099/ijs.0.020529-0
Wang, W., Chen, J., Du, K., and Xu, Z. (2004a). Morphology of spiroplasmas in the Chinese mitten crab Eriocheir sinensis associated with tremor disease. Res. Microb. 155, 630–635. doi: 10.1016/j.resmic.2004.04.010
Wang, W., Wen, B. H., Gasparich, G. E., Zhu, N. N., Rong, L. W., Chen, J. X., et al. (2004b). A spiroplasma associated with tremor disease in the Chinese mitten crab (Eriocheir sinensis). Microbiology 150, 3035–3040. doi: 10.1099/mic.0.26664-0
Wesche, S. J., Adlard, R. D., and Lester, R. J. G. (1999). Survival of spores of the oyster pathogen Marteilia sydneyi (Protozoa, Paramyxea) as assessed using fluorogenic dyes. Dis. Aquat. Organ. 36, 221–226. doi: 10.3354/dao036221
Whittington, R. J., Hick, P., Fuhrmann, M., Liu, O., and Paul-Pont, I. (2020). Removal of oyster pathogens from seawater. Environ. Int. 2020:106258. doi: 10.1016/j.envint.2020.106258
Whittington, R. J., Paul-Pont, I., Evans, O., Hick, P., and Dhand, N. K. (2018). Counting the dead to determine the source and transmission of the marine herpesvirus OsHV-1 in Crassostrea gigas. Veter. Res. 49:34.
Williams, S. L., Davidson, I. C., Pasari, J. R., Ashton, G. V., Carlton, J. T., Crafton, R. E., et al. (2013). Managing multiple vectors for marine invasions in an increasingly connected world. Bioscience 63, 952–966. doi: 10.1525/bio.2013.63.12.8
Wong, G. T. F., and Davidson, J. A. (1977). The fate of chlorine in seawater. Water Res. 11, 971–978.
Woods Hole Oceanographic Institution (WHOI) (1952). Marine fouling and its prevention. Maryland: United States Naval Institute.
Yan, S. K., and Huang, Z. G. (1993). “Biofouling of Ships in Daya Bay, China. In: The Marine Biology of the South China Sea,” in Proceedings of the First International Conference on the Marine Biology of Hong Kong and the South China Sea, (Hong Kong).
Yan, T., Yan, W., Dong, Y., Wang, H., Yan, Y., and Liang, G. (2006). Marine fouling of offshore installations in the northern Beibu Gulf of China. Int. Biodeter. Biodegr. 58, 99–105. doi: 10.1016/j.ibiod.2006.07.007
Yonemitsu, M. A., Giersch, R. M., Polo-Prieto, M., Hammel, M., Simon, A., Cremonte, F., et al. (2019). A single clonal lineage of transmissible cancer identi- fied in two marine mussel species in South America and Europe. Elife 2019:8.
Zhang, S., and Bonami, J. R. (2007). A roni−like virus associated with mortalities of the freshwater crab, Eriocheir sinensis Milne Edwards, cultured in China, exhibiting ‘sighs disease’and black gill syndrome. J. Fish Dis. 30:181. doi: 10.1111/j.1365-2761.2007.00796.x
Keywords: vessel biofouling, pathogens, mollusks, in-water cleaning, marine biosecurity
Citation: Georgiades E, Scianni C, Davidson I, Tamburri MN, First MR, Ruiz G, Ellard K, Deveney M and Kluza D (2021) The Role of Vessel Biofouling in the Translocation of Marine Pathogens: Management Considerations and Challenges. Front. Mar. Sci. 8:660125. doi: 10.3389/fmars.2021.660125
Received: 28 January 2021; Accepted: 01 April 2021;
Published: 28 April 2021.
Edited by:
Elisabeth Marijke Anne Strain, University of Tasmania, AustraliaReviewed by:
Thomas W. Therriault, Pacific Biological Station, Department of Fisheries and Oceans (Canada), CanadaGordon James Watson, University of Portsmouth, United Kingdom
Copyright © 2021 Georgiades, Scianni, Davidson, Tamburri, First, Ruiz, Ellard, Deveney and Kluza. This is an open-access article distributed under the terms of the Creative Commons Attribution License (CC BY). The use, distribution or reproduction in other forums is permitted, provided the original author(s) and the copyright owner(s) are credited and that the original publication in this journal is cited, in accordance with accepted academic practice. No use, distribution or reproduction is permitted which does not comply with these terms.
*Correspondence: Eugene Georgiades, Eugene.Georgiades@mpi.govt.nz