- 1School of Environmental Sciences, University of Guelph, Guelph, ON, Canada
- 2Department of Geography and Environmental Studies, Wilfrid Laurier University, Waterloo, ON, Canada
- 3Agriculture and Agri-Food Canada, Ottawa, ON, Canada
Liquid dairy manure treated with sulfuric acid was stored in duplicate pilot-scale storage tanks for 120 days with continuous monitoring of CH4 emissions and concurrent examination of changes in the structure of bacterial and methanogenic communities. Methane emissions were monitored at the site using laser-based Trace Gas Analyzer whereas quantitative real-time polymerase chain reaction and massively parallel sequencing were employed to study bacterial and methanogenic communities using 16S rRNA and methyl-coenzyme M Reductase A (mcrA) genes/transcripts, respectively. When compared with untreated slurries, acidification resulted in 69–84% reductions of cumulative CH4 emissions. The abundance, activity, and proportion of bacterial communities did not vary with manure acidification. However, the abundance and activity of methanogens (as estimated from mcrA gene and transcript copies, respectively) in acidified slurries were reduced by 6 and 20%, respectively. Up to 21% reduction in mcrA transcript/gene ratios were also detected in acidified slurries. Regardless of treatment, Methanocorpusculum predominated archaeal 16S rRNA and mcrA gene and transcript libraries. The proportion of Methanosarcina, which is the most metabolically-diverse methanogen, was the significant discriminant feature between acidified and untreated slurries. In acidified slurries, the relative proportions of Methanosarcina were ≤ 10%, whereas in untreated slurries, it represented up to 24 and 53% of the mcrA gene and transcript libraries, respectively. The low proportions of Methanosarcina in acidified slurries coincided with the reductions in CH4 emissions. The results suggest that reduction of CH4 missions achieved by acidification was due to an inhibition of the growth and activity of Methanosarcina species.
Introduction
Livestock production is a significant source of methane (CH4) emissions (e.g., 119.1 ± 18.2 Tg in 2011) (Wolf et al., 2017), mainly from enteric fermentation and manure management of dairy farming operations (Laubach et al., 2015; Jayasundara et al., 2016; Wolf et al., 2017). The large volumes of manure produced annually from intensive dairy farming operations areusually stored in slurry form (VanderZaag et al., 2013), which create environments conducive to CH4 production (Grant et al., 2015; Petersen, 2018). To reduce CH4 emissions from such storage systems, strategies such as reduction of aged manure (inoculants), crust development for potential aerobic CH4 oxidation, and manure acidification using sulfuric acid (H2O4) have been reported (Petersen et al., 2012; Sommer et al., 2017; Habtewold et al., 2018). Sulfuric acid-based acidification of liquid dairy manure has primarily been used to abate ammonia (NH3) emissions, but can also reduce CH4 emissions (Ottosen et al., 2009; Petersen et al., 2012; Fangueiro et al., 2015; Sommer et al., 2017). For instance, CH4 emissions from cattle slurry were reduced by 68% by acidification to pH 5.5 with H2SO4 (Sommer et al., 2017). More than 90% reduction of CH4 emissions from acidified pig slurry were also reported by Petersen et al. (2014). In fact, acidification of stored liquid dairy manure has already been implemented at farm-scale in some countries such as Denmark. In slurries, H2SO4 itself is expected to be converted to plant-available sulfate sulfur (Eriksen et al., 2008), and H2SO4 would not be found in the slurry after acidification has already occurred. However, there are no data available about the effects of manure acidification on the activities of microbial communities in stored liquid dairy manure.
In stored liquid dairy manure, complete degradation of complex organic matter involves different groups of microbial communities (hydrolytic, acidogenic, acetogenic, and methanogenic). The pH range can impact the growth and activity of these microbial groups differently, i.e., hydrolytic and acidogenic bacteria generally grow best at a pH of around 6 whereas most methanogens and acetogens have pH optima of around 7 (Lay et al., 1997; Angelidaki et al., 2003, 2011; Pind et al., 2003). Thus, slurry acidification may result in upsetting the anaerobic biodegradation processes and reduce methanogenic activity. In this study we investigated structure and activity responses of bacterial and methanogenic communities to the addition of H2SO4 to stored liquid dairy manure.
In various manure related environments, culture independent investigations of bacterial and methanogenic communities often involve using phylogenetic and/or functional gene markers (e.g., 16S rRNA and mcrA genes) (Petersen et al., 2014; Pandey et al., 2018). However, our previous study indicated that mcrA transcripts were more relevant to methane CH4 emissions than mcrA genes (Habtewold et al., 2018). Particularly with slurry acidification, where significant number of bacterial and methanogenic communities could be dormant or dead, DNA-based studies of these microbes may not reflect activities. Unlike DNA-based studies, changes in the transcriptional levels of phylogenetic and functional marker genes and transcript/functional gene ratios are strong indicators of growth and activity of microbial communities (Freitag and Prosser, 2009; Ma et al., 2012; Blagodatskaya and Kuzyakov, 2013; Wilkins et al., 2015). Hence, in the current study, we aimed to investigate abundance, activity, and diversity responses of bacterial and methanogenic communities in acidified liquid dairy manure by targeting 16S rRNA and mcrA genes and transcripts.
Materials and Methods
Methane Measurements and Manure Sampling
The study was conducted during the summer season (25 June through 23 October 2017) at the Dalhousie University’s Bio-Environmental Engineering Center (BEEC) in Truro, NS, Canada (45°45′ N, 62°50′ W). Six pilot-scale rectangular outdoor manure storage tanks covered with flow-through steady-state chambers were used. This site has been previously described by Wood et al. (2012). Fresh dairy slurry obtained from a commercial farm was loaded (10.5 m3) to each tank. Using duplicate tanks per treatment, 70% H2SO4 (1.4 L or 2.4 L L−1 slurry) or water (2.4 L L−1 slurry) were injected (with simultaneous mixing) across the depth of slurries. During storage, gas samples were drawn continuously from the inlet (ambient air) and outlet of each tank using polyethylene tubing, and CH4 concentrations were determined at the site using TGA 100A tunable diode laser trace gas analyzer (Campbell Scientific Inc., Logan, UT, United States). Methane flux (g m−2 s−1) was calculated as described by Wood et al. (2012), and emissions were then converted into daily averages.
For the microbial study, slurry samples were collected before (fresh manure) and after acidification. After acidification, manure samples were collected bi-weekly from the top (10 cm from the surface) and bottom (20 cm from floor) sections of each tank (1.8 cm). From each sampling location, nine slurry samples (on coordinates of a grid) were collected from across the surface and pooled in a clean bucket. Then, two grams subsamples (in duplicate) were collected from each pool in 15 mL Falcon tubes containing 5 mL LifeGuardTM Soil Preservation Solution (MoBio Laboratories Inc., Carlsbad, CA, United States). Samples were then transported to the lab cold and stored in a −20°C freezer until nucleic acid extractions. Based on daily CH4 fluxes, manure samples were selected after 20, 50, and 100 days of storage to assess changes in the structure of microbial communities before, during and after peak CH4 fluxes, respectively. Sub-samples of appropriate volume were also collected to analyze pH, dry matter (DM), and volatile solid (VS) contents, which were analyzed at the Nova Scotia Department of Agriculture’s Laboratory Services (Harlow Institute, Bible Hill, NS, United States) using standard methods.
Nucleic Acid Extractions and Quantitative Real-Time PCR
Slurry samples stored with LifeGuardTM Soil Preservation Solution were thawed and centrifuged (4000 × g for 10 min). Pellets were then used to co-extract total RNA and DNA using RNA PowerSoil Total RNA Isolation with DNA Elution Accessory Kits (MoBio Laboratories, Inc., Carlsbad, CA, United States) following the manufacturer’s protocol. Based on information from the manufacturer and our experience, this RNA isolation kit can be used to efficiently isolate RNA and DNA from manure samples as it does for different soil types. As there was little difference in the abundances of bacteria and methanogens between the top and bottom sections of slurries, DNA or RNA samples from these locations were pooled to have one representative sample per tank. RNA samples were reverse transcribed into complementary DNA (cDNA) using MaximaTM H Minus First Strand cDNA Synthesis Kit (Thermo ScientificTM) following the manufacturer’s protocol with few modifications. Briefly, 1 μl each of 10× dsDNase Buffer and dsDNase were added to 2 μl (0.3-1 μg) RNA, gently mixed and spun, and incubated at 37°C for 5 min in a preheated thermocycler with lid temperature adjusted to 37°C. After chilled on ice and briefly centrifuged, 4 μl Maxima cDNA H Minus Master Mix (5×) and 6 μl nuclease-free water were added, and gently mixed and centrifuged. For cDNA synthesis reactions, which were performed in a thermocycler with lid temperature adjusted to 50°C, thermal conditions were: 25°C for 10 min, 50°C for 15 min, and 85°C for 5 min. Prior to further downstream analyses, both cDNA and DNA samples were diluted and assessed for potential inhibitory effects as described previously (Habtewold et al., 2017). Diluted DNA (50×) and cDNA (100×) were then used as templates for quantitative real-time polymerase chain reaction (qPCR). Reaction ingredients, conditions, and thermal cycling of qPCR were as described by Habtewold et al. (2017). Known copies of plasmid standard curves for mcrA (10e7 to 10e1) and bacterial 16S rRNA (10e9 to 10e1 copies) genes and transcripts were prepared from Methanosarcina mazei (ATCC 43340) and a pure culture of Clostridium thermocellum, respectively. Efficiency, r2, and slope of plasmid standard curve for mcrA gene were 98.5 ± 2.8%, 0.99, and −3.34 ± 0.04, whereas for 16S rRNA gene, these values were 98.5 ± 2.7%, 0.99, and −3.36 ± 0.07, respectively. CFX Manager software version 3.1 (Bio-Rad Laboratories, Inc., Hercules, CA, United States) and GraphPad prism v.7 (GraphPad Software, Inc) were used to analyze the qPCR data.
Amplicon Library Preparation and Sequencing
Methane fluxes from all acidified slurries were very low, thus slurries treated with 2.4 L 70% H2SO4 m−3 slurry (acidified slurries) and untreated slurries were selected to study the effects of acidification on community structure of bacteria and methanogens. Polymerase chain reaction (PCR) primers (515FB-806RB) that target the V4 region of bacterial and archaeal 16S rRNA genes were used to prepare 16S rRNA gene and transcript libraries (Walters et al., 2016). To study methanogens, the gene encoding the alpha subunit of methyl coenzyme M reductase (mcrA) which is a key enzyme in methanogenesis was targeted using mlas-mod and mcrA-rev primers (Angel et al., 2011). On both 16S rRNA and mcrA gene primers, Illumina adapter sequences A and B (Supplementary Table S1) were added to the 5′-ends of the forward and reverse primers, respectively. For both genes, amplicons were prepared in two PCR steps with a total of 35 cycles. First, duplicate 25 μL PCR reactions per sample were prepared by adding 5 μL of 5X Phusion HF buffer, 0.25 μL of Thermo ScientificTM PhusionTM Hot Start II High-Fidelity DNA Polymerase (Thermo Scientific), 0.5 μL of 10 mM dNTPs (Thermo Scientific), 0.5 μL of each of the forward and reverse primers (10 μM), 2 μL of diluted DNA (10–50 ng/μL) or cDNA, and 16.25 μL nuclease free water. Thermal cycling for both genes were as follows: initial denaturation at 98°C for 3 min, followed by 25 cycles of dissociation at 98°C for 10 s, primer annealing (50°C and 55°C for 30 s, for 16S rRNA and mcrA gene/transcript, respectively), extension at 72°C for 30 s, and a final extension for 10 min. Duplicate PCR reactions were pooled and products were cleaned using silica spin columns (Wizard® SV Gel and PCR Clean-Up System; Promega) following the recommended protocol. The second step PCR was performed for 10 cycles to attach Illumina index tags to the ends of the amplicons that were obtained from the first-step PCR. For each sample, a different combination of the Index primers 1 (N7xx) and Index primers 2 (S5xx) of Illumina’s Nextera® XT DNA Library Preparation Kit (Illumina Inc., San Diego, CA, United States) were used to perform PCR. This was performed in a single 50 μL reaction mix per sample, and same proportion of reagents and thermal cycling conditions were used as the first-step PCR except the 4 μL purified amplicons template DNA. PCR products were then purified by magnetic beads (Agencourt AmPure XP; Beckman Coulter, Brea, CA, United States) and re-suspended in 25 μL. Purified PCR products were tested for correct amplicon length using gel electrophoresis and submitted to the University of Guelph Advanced Analysis Centre, Genomic Facility (Guelph, ON, Canada) for sequencing. Prior to sequencing, libraries were normalized by Sequalprep (Thermo Fisher Scientific, Hampton, NH, United States) and library quality was assessed from 6 randomly selected samples using Bioanalyzer DNA1000 chip (Agilent, Santa Clara, CA, United States). Multiplexed sample sequencing was conducted using MiSeq v3 600 cycle reagent kit (Illumina Inc., San Diego, CA, United States) producing 2 × 300 bp. Unprocessed FASTQ files were received for subsequent analysis.
Sequence Data Analysis
Raw sequence data of 16S rRNA genes and transcripts were processed and analyzed in Mothur v.1.39.5 (Schloss et al., 2009) following the recommended pipeline (Kozich et al., 2013). Briefly, forward and reverse reads of each sample were merged, target-specific primer sequences removed, and sequences were screened for ambiguity and length. Then, sequences were aligned against the Silva reference sequence (release 132), further screened for length and homopolymer, overhangs and common gaps filtered, and pre-clustered to further denoise sequencing errors. After removal of potential chimeric sequences, Mothur-formatted version of the RDP’s 16S rRNA reference (version 16) was used to classify sequences into phylotypes at 80% cut-off in which undesirable targets that might have been picked by primers were filtered. Finally, purified sequences were clustered into operational taxonomic units (OTUs) at 0.03 cut-off (97% similarity), and phylotypes of OTUs identified using the RDP’s 16S rRNA reference database. The mcrA gene and transcript sequences were processed similarly except that non-target reads and potential frameshift errors were removed or corrected using the FrameBot function of the RDP’s Functional Gene and Repository Pipeline tool (Fish et al., 2013; Wang et al., 2013). OTU-based alpha diversity (e.g., rarefaction, coverage, Chao1, and Inverse Simpson diversity estimate) and beta diversity (e.g., non-metric multidimensional scaling) analyses were performed in Mothur. Significance of differences in diversity, richness, and composition microbial community between treatments were tested in Mothur, STAMP (statistical analysis of taxonomic and functional profiles), and GraphPad prism v.7 GraphPad Software, Inc.) (Schloss et al., 2009; Parks et al., 2014).
Sequence Accessions
Raw reads of 16S rRNA and mcrA genes and transcripts have been deposited in NCBI’s short read archives as FASTQ files under the accession number SRP119447.
Results
Manure Characteristics, CH4 Flux and Microbial Abundance
Initially, the pH of fresh dairy manure used in the current study was 7.5. Twenty days after addition (and mixing) of 1.4 L or 2.4 L 70% H2SO4 per cubic meter of dairy slurry, mean pHs of slurries were 6.5 ± 0.1 and 5.9 ± 0.01, respectively (Figure 1A), while the pH of untreated slurries was 6.8 ± 0.07. After 50 days of storage, slurry pH gradually increased in all tanks by 0.35 ± 0.2. Nevertheless, pH increases in acidified slurries were small when compared with untreated control. Regardless of treatments, VS contents of slurries declined during storage (Figure 1B). Total and ammoniacal nitrogen contents (in %) of the fresh manure were 0.46 ± 0.01 and 0.18 ± 0.01, respectively. At the end of the storage period, these values were reduced to 0.37 ± 0.07 and 0.16 ± 0.02, and 0.42 ± 0.06 and 0.16 ± 0.03, in the untreated and acidified slurries, respectively. Unlike the untreated slurries, where peak CH4 fluxes (76–52 g m−2 d−1) were detected between 50 and 60 days of storage (Figure 1C), fluxes from the acidified slurries were consistently low (<10 g m−2 d−1) throughout the storage period. Addition of 1.4 L and 2.4 L 70% H2SO4 m−3 slurry resulted in 69–84% reduction of cumulative CH4 emissions when compared with untreated slurries (Figure 1D).
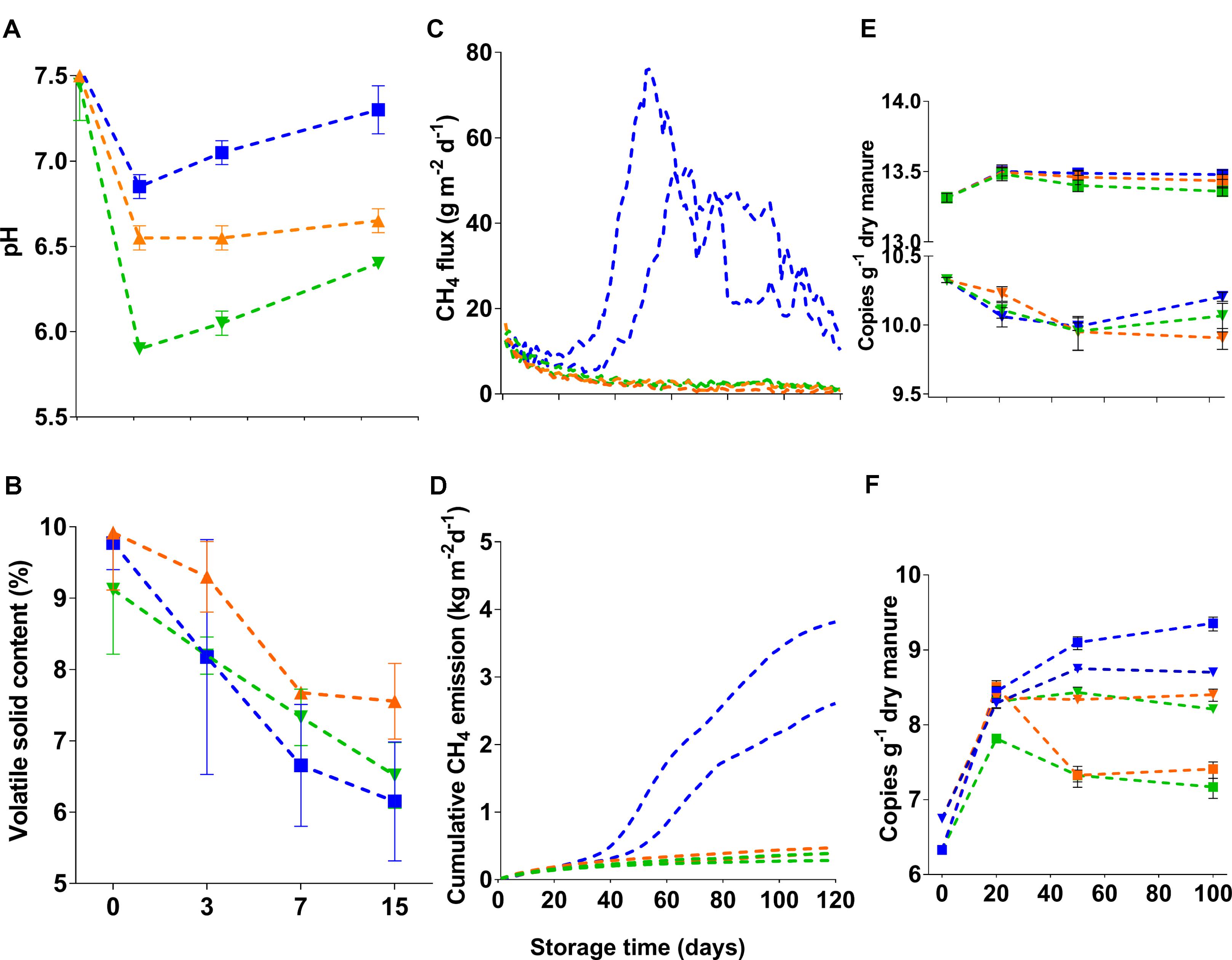
FIGURE 1. Manure characteristics (A: pH; B: volatile solid content), CH4 emissions (C: daily flux; D: cumulative emissions), and abundance of microbial communities (E: bacteria: F: methanogens). In (E,F), filled down triangle and square symbols indicate gene and transcript copies, respectively. The blue color in all figures indicates untreated manure whereas the yellow-brown and green colors indicate manures that received 1.4 L and 2.4 L 70% H2SO4, respectively.
Fresh manure (<1-day old) had a large number of bacteria, where copy numbers (Log10) of 16S rRNA genes and transcripts were 10.3 and 13.3 g−1 dry manure, respectively (Figure 1E). After 20 days of storage, the abundance of bacteria decreased in both acidified and untreated slurries. However, there were no significant differences (Kruskal–Wallis followed by Dunn’s test) in the abundance of bacteria between acidified and untreated slurries (Figure 1E). For instance, when CH4 flux peaked in untreated slurries (after 50 days of storage), differences in the copy numbers of 16S rRNA genes in acidified and untreated slurries were only about 0.4%. These small differences slightly increased (up to ∼3%) after 100 days of storage, but were not statistically significant. Thus, storage time had a greater impact on bacterial abundance than slurry acidification. Similarly, the activity of bacteria (as estimated from 16S rRNA transcript copies g−1 dry manure) showed little variation (up to 1%) with slurry acidification (Figure 1E). These results indicated that neither abundance nor activity were altered with manure acidification (pH up to 5.9).
Unlike bacteria, slurry acidification negatively affected the abundance and activity methanogenic populations. Fresh manure had 6.74 ± 0.05 copies (Log10) of mcrA genes g−1 dry manure (Figure 1F). After 20 days of storage, the mcrA gene copies showed significant increases (∼30% and ∼25% in untreated and acidified slurries, respectively; Dunn’s test, p < 0.0001). The effect of slurry acidification however was not noticeable after 20 days of storage (Figure 1F). After 50 days of storage, there were significantly lower numbers (4–4.7%; Dunn’s test, p = 0.047) of mcrA gene copies (Log10 transformed) in acidified slurries. These differences slightly increased (5–6%; Dunn’s test, p = 0.006) after 100 days of storage. The effect of slurry acidification on the activity of methanogens (estimated from mcrA transcript copies g−1 dry manure) was more significant. After 20 days of storage, slurries that received ∼2.4 L 70% H2SO4 m−3 slurry showed ∼6.5% lower copies of mcrA transcripts, while mcrA transcript/gene ratios were reduced by ∼1.7% (Supplementary Figure S2). Significant reductions (up to 32%; Dunn’s test, p < 0.0001) of mcrA transcript copies (Log10) were detected after 50 and 100 days of storage. During these time periods, mcrA transcript/gene ratios in acidified slurries were also reduced by ∼21 and 25%, respectively (Supplementary Figure S2).
Effects of Manure Acidification on the Diversity of Bacteria and Methanogens
After quality inspections of raw MiSeq sequencing data, 1863088 quality reads of 16S rRNA gene and transcript (an average of 71657 per sample) were obtained. Similarly, 524245 quality reads of mcrA gene and transcript (an average of 20163 per sample) were obtained. Diversity and community composition of bacteria and methanogens were analyzed after singletons were removed from 16S rRNA and mcrA genes and transcripts reads (Auer et al., 2017). Rarefaction plots for both gene and transcript indicated sufficient sampling efforts that might have covered most bacterial and methanogenic communities in the manure (Supplementary Figures S1a,b).
Shifts in the diversity of bacteria and methanogens due to acidification and/or storage time were shown using Inverse Simpson diversity index. Bacterial and methanogen diversity in 16S rRNA and mcrA gene libraries were higher at the beginning of storage (Table 1). However, diversities in the corresponding transcript libraries were reduced by half. These differences were consistent throughout the storage period, which might indicate the inability of several bacterial taxa from fresh manure to adapt to storage conditions. Unlike bacteria, there were significant differences (t-test, p < 0.05) in the diversity of methanogens between acidified and untreated slurries (Table 1). Diversity in mcrA gene and transcript libraries of untreated slurries also increased with storage period. These results indicated that manure acidification results in stronger impacts on methanogens when compared with bacteria.
The effects of manure acidification on the community structure of bacteria and methanogens were indicated by NMDS plots (Figures 2A,B). With a good fit of ordination (2D Stress = 0.09; r2 = 0.97), the NMDS plot did not show distinct clustering of bacterial communities from acidified and untreated slurries (Figure 2A). In line with the Inverse Simpson diversity estimates, bacterial communities in 16S rRNA gene and transcript libraries showed significant separation (AMOVA, p < 0.001) regardless of treatments. Methanogens from acidified and untreated slurries particularly after 50 days of storage showed distinct NMDS clustering patterns (2D Stress = 0.07; r2 = 0.99) which supported the diversity estimates. While the methanogens in mcrA transcript libraries of untreated slurries clustered separately from those in mcrA gene libraries (AMOVA, p < 0.05), no significant separation was observed for the acidified slurries (Figure 2B).
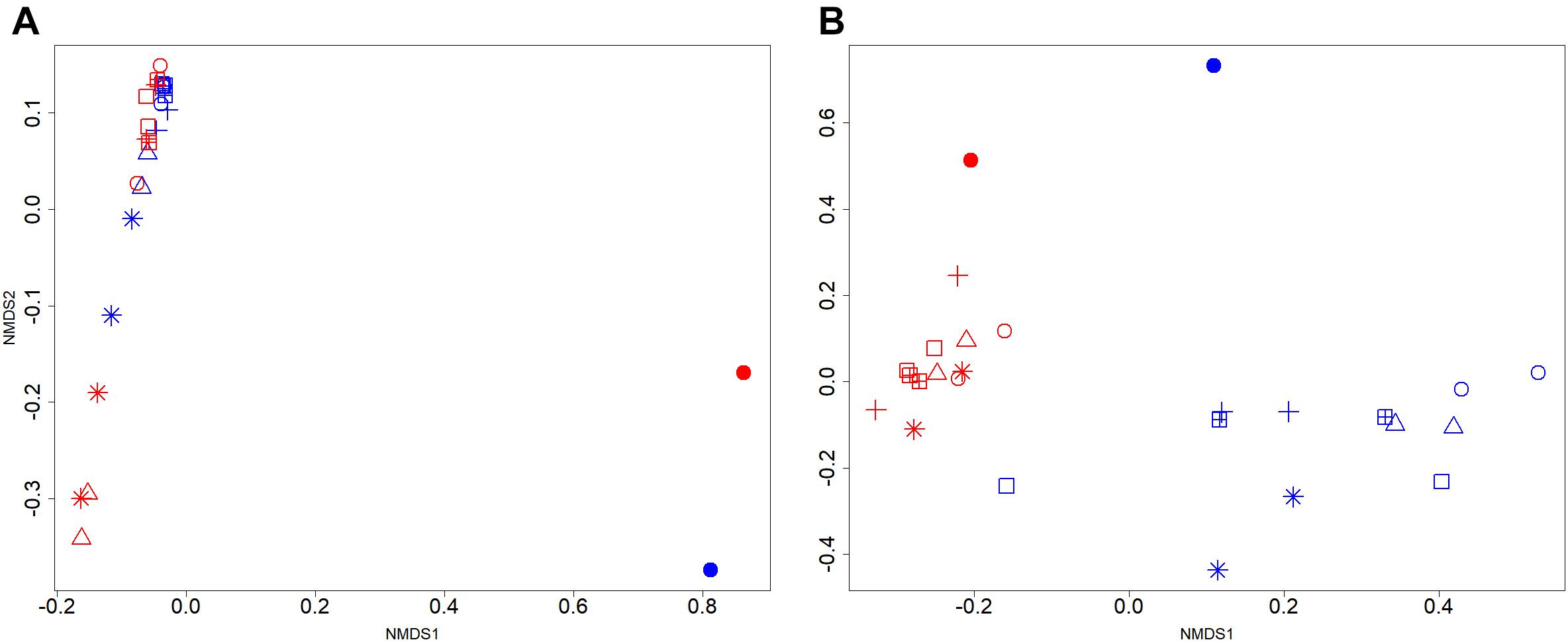
FIGURE 2. Two-dimensional non-metric multidimensional scaling (NMDS) of (A) bacterial and (B) methanogenic communities in stored liquid dairy manure. Samples from day 0, 20, 50, and 100 of untreated slurries were indicated by the filled circle, open circle, open triangle, and asterisk, respectively. Acidified slurries from day 20, 50, and 100 were indicated by the plus, square plus, and open square, respectively. Blue and red colors indicated gene and transcripts libraries, respectively.
Effects of Acidification on Relative Proportions of Bacterial and Methanogenic Phylotypes
In this study, 95.5% of the 16S rRNA gene and transcript reads were related to bacteria (Supplementary Figure S3a). In fresh manure, phylum Firmicutes and Bacteroidetes predominated (55–57% and 32–24%, respectively) 16S rRNA gene and transcript libraries (Supplementary Figure S3b). Relative proportions of the predominant bacterial phyla did not vary with slurry acidification. Regardless of treatments, 16S rRNA gene libraries from stored slurries were dominated by Firmicutes (35 ± 8%), Bacteroidetes (25 ± 4%), and Spirochaetes (15 ± 4%) whereas Firmicutes (59 ± 5%) and Bacteroidetes (21 ± 3%) predominated the 16S rRNA transcript libraries (Supplementary Figure S3b). At the genus level, Sphaerochaeta was the most abundant (15 ± 4%) bacteria in 16S rRNA gene libraries (Figure 3A). Uncultured members of Bacteroidetes (8 ± 2%), Turicibacter (6 ± 4%), and Romboutsia (6 ± 3%) were also predominant in both acidified and untreated slurries. In 16S rRNA transcript libraries of both acidified and untreated slurries, many members of Firmicutes (e.g., Romboutsia, Turicibacter, uncultured Clostridiales, Clostridium_XI, uncultured Ruminococcaceae) and uncultured Bacteroidetes accounted for 46 ± 4% and 13 ± 2%, respectively (Figure 3A).
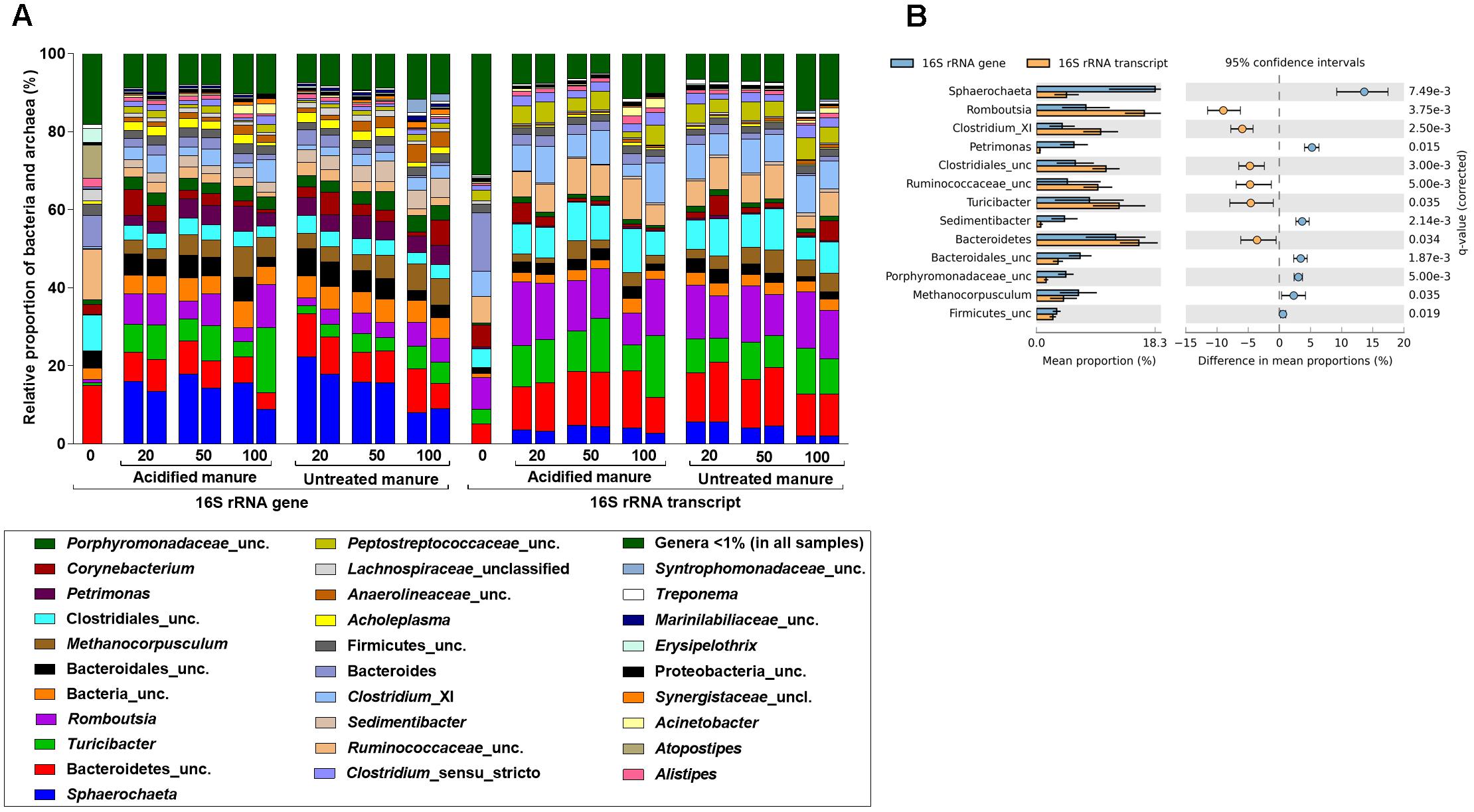
FIGURE 3. Effects of manure acidification on bacterial and archaeal phylotypes as indicated by (A) relative proportions of abundant genera in fresh, acidified and untreated slurries (B) extended error bar plots illustrating significantly abundant bacteria (Effect size = 2, White’s non-parametric t-test with Benjamini–Hochberg multiple test correction, q-values < 0.05) in acidified and untreated slurries. Numbers on the X axis indicate storage time in days. Except fresh manure, all have biological replicates (n = 2).
Analysis of differences in mean proportions of 16S rRNA gene and transcript reads between acidified and untreated slurries (White’s non-parametric t-test with Bonferroni correction, CI = 95%, α = 0.05) indicated that manure acidification with H2SO4 did not alter the composition of bacterial communities. Regardless of treatments, 16S rRNA gene and transcript communities were significantly different (White’s non-parametric t-test, p = 0.015; Figure 3B), which was in line with the NMDS analysis. Genera from different bacterial phyla (e.g., Spirochaeta, Petrimonas, and Sedimentibacter) and members of the Firmicutes (e.g., Romboutsia, Clostridium_XI, and uncultured members Ruminococcaceae) were represented differently in the 16S rRNA gene and transcript communities.
Archaea accounted for 4.5% of 16S rRNA gene and transcript reads (Supplementary Figure S3a), and all were methanogens. The most abundant genus in fresh manure was Methanobrevibacter (76%), but Methanocorpusculum was predominant (92 ± 1% and 86 ± 2% in archaeal 16S rRNA gene and transcript libraries, respectively) in stored slurries of all treatments (Figure 4). While the proportion of Methanosarcina in 16S rRNA gene and transcript libraries of untreated slurries gradually increased (up to 5 and 18%, respectively), its proportions in acidified slurries was consistently below 1%. Thus, Methanosarcina seemed strongly inhibited by manure acidification.
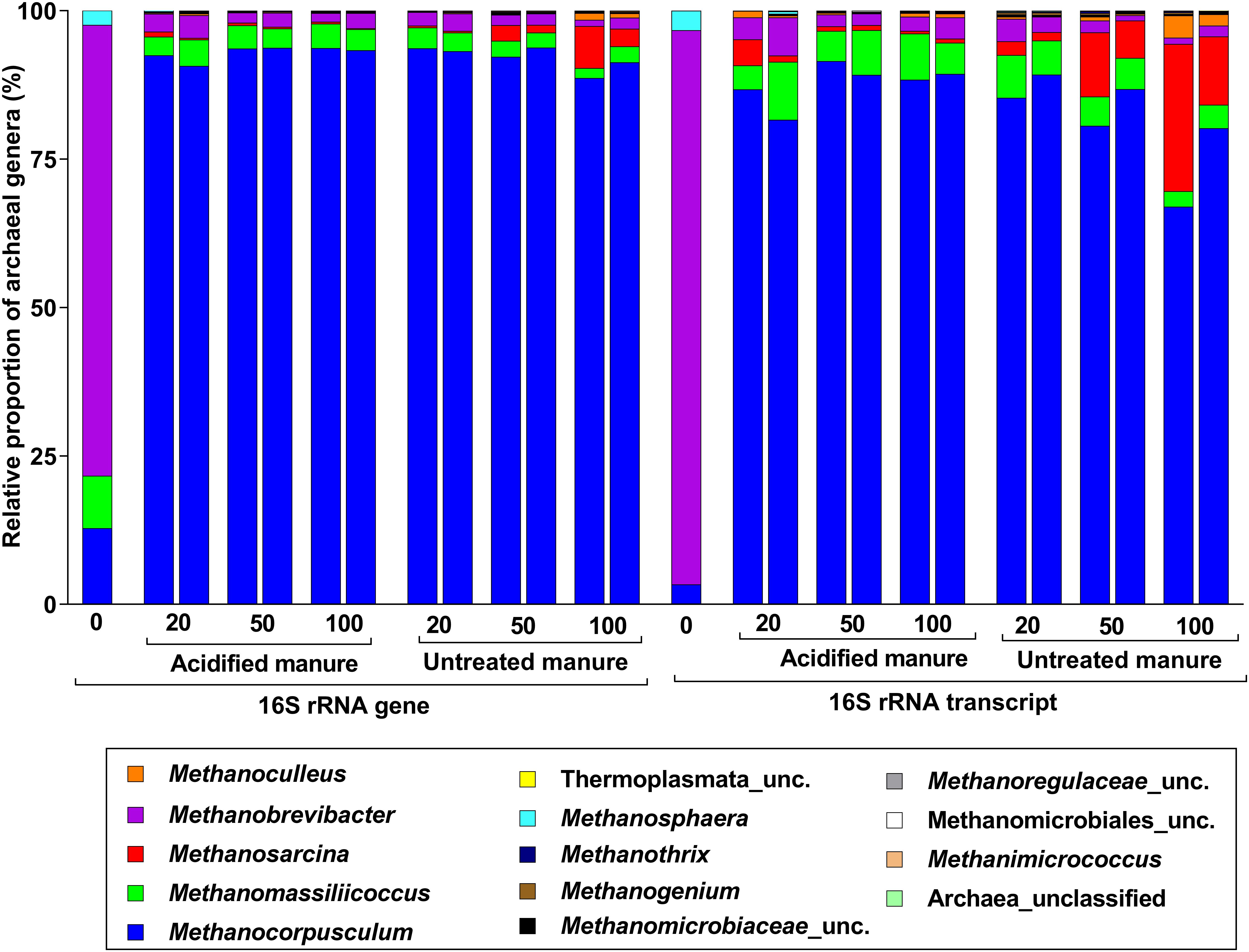
FIGURE 4. Taxonomic distribution of archaeal phylotypes identified from 16S rRNA gene and transcript reads. Numbers on the X-axis show storage time in days. Except fresh manure, all have biological replicates (n = 2).
Like in archaeal 16S rRNA gene libraries, Methanobrevibacter predominated the mcrA gene library from fresh manure (Figure 5A). Although Methanocorpusculum represented < 1% of the methanogens in fresh manure, it represented 83 ± 3% of the mcrA gene and transcript reads from acidified slurries. Methanocorpusculum was also dominant in untreated slurries but gradually declined (84 to 59% and 84 to 46% in the mcrA gene and transcript libraries, respectively) with storage time. In contrast, the relative proportion of Methanosarcina, increased (4 to 25% and 13 to 41% in the mcrA gene and transcript libraries, respectively) in untreated slurries, but in acidified slurries the population remained stable. Increased amounts of CH4 emitted from untreated slurries coincided with the increased abundance of Methanosarcina.
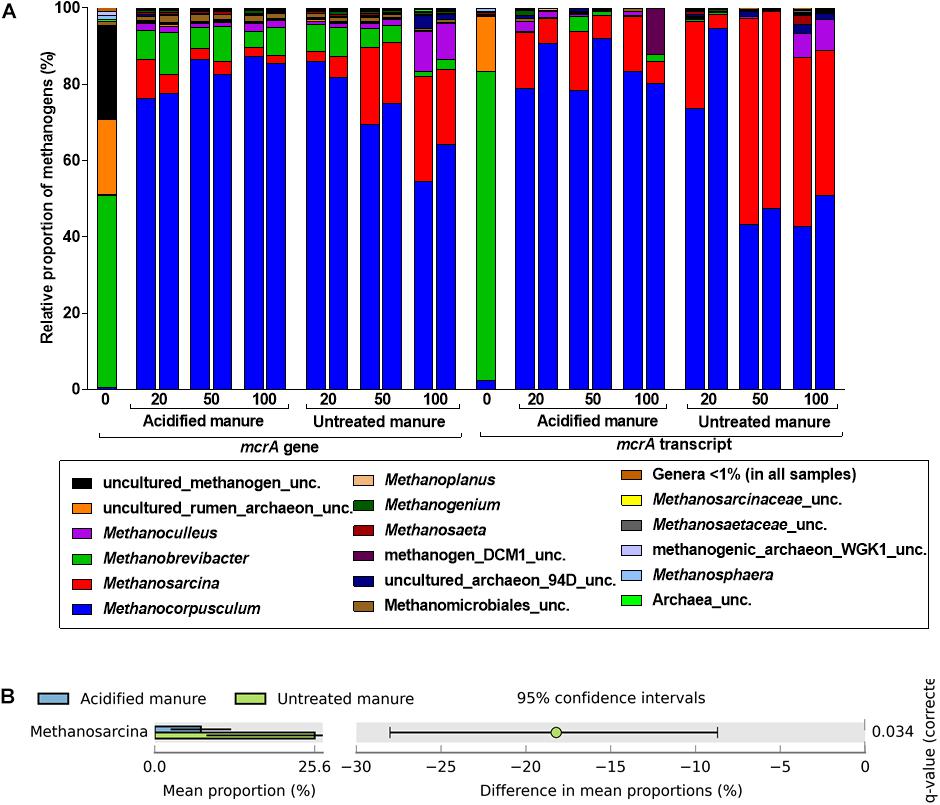
FIGURE 5. Effects of manure acidification on methanogens as indicated by (A) a stacked bar showing relative proportions of methanogenic genera in fresh, acidified and untreated slurries (B) extended error bar plots illustrating significantly abundant methanogens (Effect size = 2, White’s non-parametric t-test with Benjamini–Hochberg multiple test correction, q-values < 0.05) in acidified and untreated slurries. Numbers on the X axis indicate storage time in days. Except fresh manure, all have biological replicates (n = 2).
Analysis of differences in mean proportions of mcrA gene reads between acidified and untreated slurries indicated that Methanosarcina (p = 0.05) was differentially enriched in untreated slurries (Figure 5B), indicating the negative impacts of manure acidification on methanogens related to the genus Methanosarcina.
Discussion
Stored liquid dairy manure is a point source of CH4. Studies have demonstrated that H2SO4-based acidification of liquid dairy manure (reducing pH to ∼5.5) can reduce CH4 emissions from 67 to 90% (Petersen et al., 2012, 2014; Misselbrook et al., 2016; Sommer et al., 2017). In line with these studies, 69–84% reductions of cumulative CH4 emissions were detected in this study after acidification of dairy manure to pH 6.5 and 5.9. Thus, small changes in slurry pH were able to disrupt methanogenesis that has pH optima around 7 (Lay et al., 1997; Liu et al., 2008; Weiland, 2010; Mao et al., 2017); however, reduction in CH4 emissions could also be related to the toxicity of hydrogen sulfide that could be accumulated as a result of potential sulfate reduction (Petersen et al., 2012). Throughout the storage period, CH4 fluxes from acidified slurries remained low, consistent with levels observed during the lag phase (the first 20 days of storage, Figure 1C). The lag phase observed in the current study was also observed in our previous studies that did not involve acidification (Habtewold et al., 2018). A previous study from our lab has linked shifts in methanogens to methane emissions (Habtewold et al., 2018), therefore we predicted that the communities would shift after acidification. Using qPCR-based quantification and deep sequencing (Illumina MiSeq) of phylogenetic and functional marker genes and transcripts, we demonstrated that slurry acidification (to pH 5.9) did not affect the community structure of most anaerobically-degrading microorganisms except methanogens closely related to the genus Methanosarcina. As major players in CH4 production (Conrad, 1999), impacts on methanogens related to Methanosarcina can have a drastic effect on methanogenesis and CH4 emissions.
With slurry acidification (mean pHs 6.5 and 5.9), shifts in the abundance and activities of bacterial communities, as estimated from 16S rRNA gene and transcript copies, were not significant. Thus, acidification might have little effect on growth and activities of bacterial communities involved in the anaerobic degradation of organic matter (e.g., hydrolytic, acidogenic, and acetogenic bacteria) in manure (Lin et al., 2013; Kuruti et al., 2017). As acidification may reduce aggregation of slurry particles (Fangueiro et al., 2015; Gomez-Munoz et al., 2016; Regueiro et al., 2016), substrate availability for hydrolytic and acidogenic bacteria in slurries may increase. Gradual reductions in the volatile solids contents of both acidified and untreated slurries indicated that these communities were active. Microbial consumption of volatile solids in manure typically increases the amount of organic acids (e.g., acetic, propionic, and butyric acids) and methanogenic substrates (e.g., CO2, H2, acetate, formate, and alcohol). Thus, pH reductions observed during the first 20 days of storage (regardless of treatment) might be related to accumulation of organic acids. Although pH reductions due to organic acid accumulation might be obscured in acidified slurries, reductions in total solids contents in these slurries might indicate microbial activities. Regardless of treatments, slurry pH gradually increased after 50 days of storage which was in line with other studies (Patni and Jui, 1985; Sommer et al., 2017). However, pH increases (often due to consumption of organic acids by acetogens and methanogens) in acidified slurries were lower when compared with untreated slurries. These small changes in pH coincided with low CH4 flux from acidified slurries, indicating negative impacts of acidification with H2SO4 on methanogens.
Acidification had little effect on the abundance of methanogenic populations. This was in line with a study by Petersen et al. (2014) where the abundance of methanogens in pig slurry did not shift with slurry acidification (pH down to 5.5). However, the authors detected more than 90% reductions in CH4 emission which indicate the negative impacts of slurry acidifications with H2SO4 on methanogenic processes. Ottosen et al. (2009) also detected significant reductions (>98%) in microbial processes (oxygen consumption rate, methanogenesis and sulfate reduction) in acidified pig slurry. As DNA-based studies of mcrA genes provide information about all methanogens (active, dormant, and dead), in this study we used instead mRNA of the mcrA genes (mcrA transcript) to specifically study changes in physiological status of methanogens and methanogenic processes. Unlike population abundance, the reduced copy numbers of mcrA transcripts in acidified slurries with negligible CH4 emissions might reflect the negative effect of manure acidification on the activities of methanogens. However, some or most methanogens might still grow and function in acidified slurries as the abundance and activities of methanogens in it were higher when compared with fresh manure. This would account for the residual methane emission observed.
With little impacts of acidification on the abundance and activity of bacteria, accumulated intermediary compounds including propionate, butyrate, and valerate could be converted into acetate by the acetogens (Demirel and Scherer, 2008), making stored liquid dairy manure rich in acetate (Barret et al., 2013; Habtewold et al., 2018). Although acetoclastic methanogenesis (using acetate as substrate) is the major contributor of CH4 produced in many environments (Conrad, 1999), CH4 production in environments with high concentration of acetate has been found to drastically reduce as pH decline (Van Kessel and Russell, 1996), although the exact mechanism is not yet clear.
Consistent with the qPCR data, the diversity and relative proportions of bacterial communities were not altered with slurry acidification (to pH 5.9). Regardless of treatments, Sphaerochaeta was predominant in the 16S rRNA gene libraries. These bacteria are enriched with fermentation and carbohydrate metabolism genes (Caro-Quintero et al., 2012), but it is unclear why they represented lower proportions in the 16S rRNA transcript libraries where several fermentative bacteria (e.g., Turicibacter, Bacteriodetes, and Romboutsia) (Bosshard et al., 2002; Thomas et al., 2011; Gerritsen et al., 2017) were predominant. The abundance of these bacteria, particularly in the 16S rRNA transcript libraries of acidified slurries, might indicate availability of methanogenic substrates. Regardless of treatments, methanogens closely related to the genus Methanocorpusculum that are known to perform hydrogenotrophic methanogenesis (reducing CO2 to CH4 using hydrogen) predominated the archaeal 16S rRNA and mcrA gene and transcript libraries. This was in line with our previous pilot-scale studies conducted using manure imported from the same commercial farm (Habtewold et al., 2017, 2018). However, CH4 emissions were significant only in untreated slurries where the proportion of methanogens closely related to the genus Methanosarcina had significantly increased. In contrast to many other methanogens, Methanosarcina has been reported to grow under high concentrations of ammonia and VFA (Demirel and Scherer, 2008). However, the current study indicated that these methanogens were apparently impacted by the acidification with H2SO4 and perhaps by products of sulfate reduction (e.g., H2S). Compared to acidified slurries, the predominance of Methanosarcina was high in untreated slurries which coincided with increased CH4 emissions. Methanosarcina species are metabolically the most diverse and have higher efficiency in CH4 production (e.g., 3× when glucose is used as substrate) when compared with Methanocorpusculum (Conrad, 1999; Kotsyurbenko et al., 2004), thus any effect on these methanogens might result in significant reduction of CH4 production.
In stored liquid manure, reductions in CH4 emissions might also be related to potential methanotrophy, which is presumed to occur in the surface crusts of slurries where oxygen is freely available for methanotrophs (Petersen and Ambus, 2006). In the current study, no crust was formed, and no known methanotroph was detected in both the 16S rRNA gene and transcript libraries of all treatments. Thus, the contribution of methanotrophy to the reduction of CH4 emissions detected in the current study were less likely.
With the use of H2SO4 for manure acidification, slurries can be enriched with sulfate which is an important substrate for sulfate-reducing bacteria that have high affinity to available hydrogen (Kristjansson and Schönheit, 1983). Although the relative proportions of sulfate-reducers detected in the current study (e.g., Desulfatibacillum, Desulforhopalus, Desulfuromonas, and Desulfobulbus) were low, together with potential homoacetogenic bacteria (e.g., Acetobacterium and Blautia), they might still compete hydrogenotrophic methanogens for available substrates (Weijma et al., 2002). Methanogens related to the genus Methanosarcina can perform all three pathways of methanogenesis (hydrogenotrophic, acetoclastic, and methylotrophic), thus may compete favorably by changing substrates. Although sulfate-reducing bacteria can also compete for acetate, this substrate is highly abundant in stored liquid dairy manure (Barret et al., 2013; Habtewold et al., 2018), and acetate consumption rates in methanogens are relatively higher when compared to sulfate reducers (Bhattacharya et al., 1996). Hydrogen sulfide, which could be accumulated in slurries as a result potential sulfate reduction, might also suppress the activities of methanogens except Methanosarcina (Demirel and Scherer, 2008). Thus, differential enrichment of Methanosarcina in untreated slurries indicated manure acidification with H2SO4 had more impact on these methanogens when compared to Methanocorpusculum.
Conclusion
H2SO4-based acidification of stored liquid dairy manure (mean pH 6.5 and 5.9) could reduce cumulative CH4 emissions by 76 ± 7% and 78 ± 6%, respectively. Slurry acidification (pH down to 5.9) with H2SO4 coincided with significant reduction of VS contents of slurries in all treatments, but did not significantly impact the abundance, activity or community structure of bacteria. Regardless of treatments, Methanocorpusculum was the predominant methanogenic genus. Methanosarcina, while representing a minor proportion of the methanogens in this dairy slurry, was relatively lower in acidified slurries, and this coincided with significant reductions in CH4 emissions. Thus, we propose that manure acidification with H2SO4 reduced CH4 emissions by inhibiting growth and activities of Methanosarcina, the most metabolically diverse methanogen.
Author Contributions
All the authors were involved in the planning of the work and revision of the manuscript. JH conducted the molecular work, data analysis, interpretations, and prepared the manuscript with the guidance of KD and RG.
Conflict of Interest Statement
The authors declare that the research was conducted in the absence of any commercial or financial relationships that could be construed as a potential conflict of interest.
Acknowledgments
The authors would like to acknowledge the Ontario Ministry of Agriculture, Food and Rural Affairs for financial support.
Supplementary Material
The Supplementary Material for this article can be found online at: https://www.frontiersin.org/articles/10.3389/fmicb.2018.02806/full#supplementary-material
References
Angel, R., Matthies, D., and Conrad, R. (2011). Activation of methanogenesis in arid biological soil crusts despite the presence of oxygen. PLoS One 6:e20453. doi: 10.1371/journal.pone.0020453
Angelidaki, I., Ellegaard, L., and Ahring, B. K. (2003). Applications of the anaerobic digestion process. Adv. Biochem. Eng. Biotechnol. 82, 1–33. doi: 10.1007/3-540-45838-7_1
Angelidaki, I., Karakashev, D., Batstone, D. J., Plugge, C. M., and Stams, A. J. (2011). Biomethanation and its potential. Methods Enzymol. 494, 327–351. doi: 10.1016/B978-0-12-385112-3.00016-0
Auer, L., Mariadassou, M., O’Donohue, M., Klopp, C., and Hernandez-Raquet, G. (2017). Analysis of large 16S rRNA Illumina data sets: impact of singleton read filtering on microbial community description. Mol. Ecol. Resour. 17, e122–e132. doi: 10.1111/1755-0998.12700
Barret, M., Gagnon, N., Topp, E., Masse, L., Masse, D. I., and Talbot, G. (2013). Physico-chemical characteristics and methanogen communities in swine and dairy manure storage tanks: spatio-temporal variations and impact on methanogenic activity. Water Res. 47, 737–746. doi: 10.1016/j.watres.2012.10.047
Bhattacharya, S. K., Uberoi, V., and Dronamraju, M. M. (1996). Interaction between acetate fed sulfate reducers and methanogens. Water Res. 30, 2239–2246. doi: 10.1016/0043-1354(95)00238-3
Blagodatskaya, E., and Kuzyakov, Y. (2013). Active microorganisms in soil: critical review of estimation criteria and approaches. Soil Biol. Biochem. 67, 192–211. doi: 10.1016/j.soilbio.2013.08.024
Bosshard, P. P., Zbinden, R., and Altwegg, M. (2002). Turicibacter sanguinis gen. nov., sp. nov., a novel anaerobic, gram-positive bacterium. Int. J. Syst. Evol. Microbiol. 52(Pt 4), 1263–1266. doi: 10.1099/00207713-52-4-1263
Caro-Quintero, A., Ritalahti, K. M., Cusick, K. D., Löffler, F. E., and Konstantinidis, K. T. (2012). The chimeric genome of sphaerochaeta: nonspiral spirochetes that break with the prevalent dogma in spirochete biology. mBIO 3:e0025-12. doi: 10.1128/mBio.00025-12
Conrad, R. (1999). Contribution of hydrogen to methane production and control of hydrogen concentrations in methanogenic soils and sediments. FEMS Microbiol. Ecol. 28, 193–202. doi: 10.1111/j.1574-6941.1999.tb00575.x
Demirel, B., and Scherer, P. (2008). The roles of acetotrophic and hydrogenotrophic methanogens during anaerobic conversion of biomass to methane: a review. Rev. Environ. Sci. Biotechnol. 7:173. doi: 10.1007/s11157-008-9131-1
Eriksen, J., Sørensen, P., and Elsgaard, L. (2008). The fate of sulfate in acidified pig slurry during storage and following application to cropped soil. J. Environ. Qual. 37, 280–286. doi: 10.2134/jeq2007.0317
Fangueiro, D., Pereira, J., Bichana, A., Surgy, S., Cabral, F., and Coutinho, J. (2015). Effects of cattle-slurry treatment by acidification and separation on nitrogen dynamics and global warming potential after surface application to an acidic soil. J. Environ. Manage. 162, 1–8. doi: 10.1016/j.jenvman.2015.07.032
Fish, J. A., Chai, B. L., Wang, Q., Sun, Y. N., Brown, C. T., Tiedje, J. M., et al. (2013). FunGene: the functional gene pipeline and repository. Front. Microbiol. 4:291. doi: 10.3389/Fmicb.2013.00291
Freitag, T. E., and Prosser, J. I. (2009). Correlation of methane production and functional gene transcriptional activity in a peat soil. Appl. Environ. Microbiol. 75, 6679–6687. doi: 10.1128/AEM.01021-09
Gerritsen, J., Hornung, B., Renckens, B., van Hijum, S., Martins Dos Santos, V. A. P., Rijkers, G. T., et al. (2017). Genomic and functional analysis of Romboutsia ilealis CRIB(T) reveals adaptation to the small intestine. PeerJ 5:e3698. doi: 10.7717/peerj.3698
Gomez-Munoz, B., Case, S. D., and Jensen, L. S. (2016). Pig slurry acidification and separation techniques affect soil N and C turnover and N2O emissions from solid, liquid and biochar fractions. J. Environ. Manage. 168, 236–244. doi: 10.1016/j.jenvman.2015.12.018
Grant, R. H., Boehm, M. T., and Bogan, B. W. (2015). Methane and carbon dioxide emissions from manure storage facilities at two free-stall dairies. Agric. Meteorol. 213, 102–113. doi: 10.1016/j.agrformet.2015.06.008
Habtewold, J., Gordon, R., Sokolov, V., VanderZaag, A., Wagner-Riddle, C., and Dunfield, K. (2018). Targeting bacteria and methanogens to understand the role of residual slurry as an inoculant in stored liquid dairy manure. Appl. Environ. Microbiol. 84:e02830–17. doi: 10.1128/AEM.02830-17
Habtewold, J., Gordon, R. J., Wood, J. D., Wagner-Riddle, C., VanderZaag, A. C., and Dunfield, K. E. (2017). Dairy manure total solid levels impact CH4 Flux and abundance of methanogenic archaeal communities. J. Environ. Qual. 46, 232–236. doi: 10.2134/jeq2016.11.0451
Jayasundara, S., Ranga Niroshan Appuhamy, J. A. D., Kebreab, E., and Wagner-Riddle, C. (2016). Methane and nitrous oxide emissions from canadian dairy farms and mitigation options: an updated review. Can. J. Anim. Sci. 96, 306–331. doi: 10.1139/cjas-2015-0111
Kotsyurbenko, O. R., Chin, K. J., Glagolev, M. V., Stubner, S., Simankova, M. V., Nozhevnikova, A. N., et al. (2004). Acetoclastic and hydrogenotrophic methane production and methanogenic populations in an acidic west-siberian peat bog. Environ. Microbiol. 6, 1159–1173. doi: 10.1111/j.1462-2920.2004.00634.x
Kozich, J. J., Westcott, S. L., Baxter, N. T., Highlander, S. K., and Schloss, P. D. (2013). Development of a dual-index sequencing strategy and curation pipeline for analyzing amplicon sequence data on the MiSeq illumina sequencing platform. Appl. Environ. Microbiol. 79, 5112–5120. doi: 10.1128/Aem.01043-13
Kristjansson, J. K., and Schönheit, P. (1983). Why do sulfate-reducing bacteria outcompete methanogenic bacteria for substrates? Oecologia 60, 264–266. doi: 10.1007/BF00379530
Kuruti, K., Nakkasunchi, S., Begum, S., Juntupally, S., Arelli, V., and Anupoju, G. R. (2017). Rapid generation of volatile fatty acids (VFA) through anaerobic acidification of livestock organic waste at low hydraulic residence time (HRT). Bioresour. Technol. 238, 188–193. doi: 10.1016/j.biortech.2017.04.005
Laubach, J., Heubeck, S., Pratt, C., Woodward, K. B., Guieysse, B., van der Weerden, T. J., et al. (2015). Review of greenhouse gas emissions from the storage and land application of farm dairy effluent. N. Z. J. Agric. Res. 58, 203–233. doi: 10.1080/00288233.2015.1011284
Lay, J. J., Li, Y. Y., and Noike, T. (1997). Influences of pH and moisture content on the methane production in high-solids sludge digestion. Water Res. 31, 1518–1524. doi: 10.1016/S0043-1354(96)00413-7
Lin, L., Wan, C. L., Liu, X., Lee, D. J., Lei, Z. F., Zhang, Y., et al. (2013). Effect of initial pH on mesophilic hydrolysis and acidification of swine manure. Bioresour. Technol. 136, 302–308. doi: 10.1016/j.biortech.2013.02.106
Liu, C. F., Yuan, X. Z., Zeng, G. M., Li, W. W., and Li, J. (2008). Prediction of methane yield at optimum pH for anaerobic digestion of organic fraction of municipal solid waste. Bioresour. Technol. 99, 882–888. doi: 10.1016/j.biortech.2007.01.013
Ma, K., Conrad, R., and Lu, Y. H. (2012). Responses of methanogen mcrA genes and their transcripts to an alternate Dry/Wet cycle of paddy field soil. Appl. Environ. Microbiol. 78, 445–454. doi: 10.1128/Aem.06934-11
Mao, C. L., Zhang, T., Wang, X. J., Feng, Y. Z., Ren, G. X., and Yang, G. H. (2017). Process performance and methane production optimizing of anaerobic co-digestion of swine manure and corn straw. Sci. Rep. 7:9379. doi: 10.1038/S41598-017-09977-6
Misselbrook, T., Hunt, J., Perazzolo, F., and Provolo, G. (2016). Greenhouse gas and ammonia emissions from slurry storage: impacts of temperature and potential mitigation through covering (Pig Slurry) or acidification (Cattle Slurry). J. Environ. Qual. 45, 1520–1530. doi: 10.2134/jeq2015.12.0618
Ottosen, L. D. M., Poulsen, H. V., Nielsen, D. A., Finster, K., Nielsen, L. P., and Revsbech, N. P. (2009). Observations on microbial activity in acidified pig slurry. Bios. Eng. 102, 291–297. doi: 10.1016/j.biosystemseng.2008.12.003
Pandey, P., Chiu, C., Miao, M., Wang, Y., Settles, M., del Rio, N. S., et al. (2018). 16S rRNA analysis of diversity of manure microbial community in dairy farm environment. PLoS One 13:e0190126. doi: 10.1371/journal.pone.0190126
Parks, D. H., Tyson, G. W., Hugenholtz, P., and Beiko, R. G. (2014). STAMP: statistical analysis of taxonomic and functional profiles. Bioinformatics 30, 3123–3124. doi: 10.1093/bioinformatics/btu494
Patni, N. K., and Jui, P. Y. (1985). Volatile fatty-acids in stored dairy-cattle slurry. Agric. Was. 13, 159–178. doi: 10.1016/0141-4607(85)90031-9
Petersen, S. O. (2018). Greenhouse gas emissions from liquid dairy manure: prediction and mitigation. J. Dairy Sci. 101, 6642–6654. doi: 10.3168/jds.2017-13301
Petersen, S. O., and Ambus, P. (2006). Methane oxidation in pig and cattle slurry storages, and effects of surface crust moisture and methane availability. Nutr. Cycl. Agroecosys. 74, 1–11. doi: 10.1007/s10705-005-3822-6
Petersen, S. O., Andersen, A. J., and Eriksen, J. (2012). Effects of cattle slurry acidification on ammonia and methane evolution during storage. J. Environ. Qual. 41, 88–94. doi: 10.2134/jeq2011.0184
Petersen, S. O., Hojberg, O., Poulsen, M., Schwab, C., and Eriksen, J. (2014). Methanogenic community changes, and emissions of methane and other gases, during storage of acidified and untreated pig slurry. J. Appl. Microbiol. 117, 160–172. doi: 10.1111/jam.12498
Pind, P. F., Angelidaki, I., Ahring, B. K., Stamatelatou, K., and Lyberatos, G. (2003). Monitoring and control of anaerobic reactors. Adv. Biochem. Eng. Biotechnol. 82, 135–182. doi: 10.1007/3-540-45838-7_4
Regueiro, I., Pociask, M., Coutinho, J., and Fangueiro, D. (2016). Animal slurry acidification affects particle size distribution and improves separation efficiency. J. Environ. Qual. 45, 1096–1103. doi: 10.2134/jeq2015.07.0403
Schloss, P. D., Westcott, S. L., Ryabin, T., Hall, J. R., Hartmann, M., Hollister, E. B., et al. (2009). Introducing mothur: open-source, platform-independent, community-supported software for describing and comparing microbial communities. Appl. Environ. Microbiol. 75, 7537–7541. doi: 10.1128/Aem.01541-09
Sommer, S. G., Clough, T. J., Balaine, N., Hafner, S. D., and Cameron, K. C. (2017). Transformation of organic matter and the emissions of methane and ammonia during storage of liquid manure as affected by acidification. J. Environ. Qual. 46, 514–521. doi: 10.2134/jeq2016.10.0409
Thomas, F., Hehemann, J. H., Rebuffet, E., Czjzek, M., and Michel, G. (2011). Environmental and gut bacteroidetes: the food connection. Front. Microbiol. 2:93. doi: 10.3389/fmicb.2011.00093
Van Kessel, J. A. S., and Russell, J. B. (1996). The effect of pH on ruminal methanogenesis. FEMS Microbiol. Ecol. 20, 205–210. doi: 10.1016/0168-6496(96)00030-X
VanderZaag, A. C., MacDonald, J. D., Evans, L., Verge, X. P. C., and Desjardins, R. L. (2013). Towards an inventory of methane emissions from manure management that is responsive to changes on canadian farms. Environ. Res. Lett. 8:e035008. doi: 10.1088/1748-9326/8/3/035008
Walters, W., Hyde, E. R., Berg-Lyons, D., Ackermann, G., Humphrey, G., Parada, A., et al. (2016). Improved bacterial 16S rRNA gene (V4 and V4-5) and fungal internal transcribed spacer marker gene primers for microbial community surveys. mSystems 1:e00009–15. doi: 10.1128/mSystems.00009-15
Wang, Q., Quensen, J. F., Fish, J. A., Lee, T. K., Sun, Y. N., Tiedje, J. M., et al. (2013). Ecological patterns of nifH genes in four terrestrial climatic zones explored with targeted metagenomics using framebot, a new informatics tool. mBio 4:e592–13. doi: 10.1128/mBio.00592-13
Weijma, J., Gubbels, F., Hulshoff Pol, L. W., Stams, A. J., Lens, P., and Lettinga, G. (2002). Competition for H2 between sulfate reducers, methanogens and homoacetogens in a gas-lift reactor. Water Sci. Technol. 45, 75–80. doi: 10.2166/wst.2002.0294
Weiland, P. (2010). Biogas production: current state and perspectives. Appl. Microbiol. Biotechnol. 85, 849–860. doi: 10.1007/s00253-009-2246-7
Wilkins, D., Lu, X. Y., Shen, Z. Y., Chen, J. P., and Lee, P. K. H. (2015). Pyrosequencing of mcrA and archaeal 16S rRNA genes reveals diversity and substrate preferences of methanogen communities in anaerobic digesters. Appl. Environ. Microbiol. 81, 604–613. doi: 10.1128/Aem.02566-14
Wolf, J., Asrar, G. R., and West, T. O. (2017). Revised methane emissions factors and spatially distributed annual carbon fluxes for global livestock. Carbon Balance Manag. 12:16. doi: 10.1186/s13021-017-0084-y
Keywords: dairy manure, greenhouse gas, manure acidification, methane, methanogens
Citation: Habtewold J, Gordon R, Sokolov V, VanderZaag A, Wagner-Riddle C and Dunfield K (2018) Reduction in Methane Emissions From Acidified Dairy Slurry Is Related to Inhibition of Methanosarcina Species. Front. Microbiol. 9:2806. doi: 10.3389/fmicb.2018.02806
Received: 21 August 2018; Accepted: 31 October 2018;
Published: 20 November 2018.
Edited by:
Adrian Ho, Leibniz Universität Hannover, GermanyReviewed by:
Søren O. Petersen, Aarhus University, DenmarkSang Yoon Kim, Sunchon National University, South Korea
Copyright © 2018 Habtewold, Gordon, Sokolov, VanderZaag, Wagner-Riddle and Dunfield. This is an open-access article distributed under the terms of the Creative Commons Attribution License (CC BY). The use, distribution or reproduction in other forums is permitted, provided the original author(s) and the copyright owner(s) are credited and that the original publication in this journal is cited, in accordance with accepted academic practice. No use, distribution or reproduction is permitted which does not comply with these terms.
*Correspondence: Kari Dunfield, dunfield@uoguelph.ca