- 1Department of Botany, St. Joseph’s University, Bangalore, KA, India
- 2Institute of Sericulture and Tea, Zhejiang Academy of Agricultural Sciences, Hangzhou, Zhejiang, China
- 3Independent Researcher, Bangalore, KA, India
Climate change has increased the overall impact of abiotic stress conditions such as drought, salinity, and extreme temperatures on plants. Abiotic stress adversely affects the growth, development, crop yield, and productivity of plants. When plants are subjected to various environmental stress conditions, the balance between the production of reactive oxygen species and its detoxification through antioxidant mechanisms is disturbed. The extent of disturbance depends on the severity, intensity, and duration of abiotic stress. The equilibrium between the production and elimination of reactive oxygen species is maintained due to both enzymatic and non-enzymatic antioxidative defense mechanisms. Non-enzymatic antioxidants include both lipid-soluble (α-tocopherol and β-carotene) and water-soluble (glutathione, ascorbate, etc.) antioxidants. Ascorbate peroxidase (APX), superoxide dismutase (SOD), catalase (CAT), and glutathione reductase (GR) are major enzymatic antioxidants that are essential for ROS homeostasis. In this review, we intend to discuss various antioxidative defense approaches used to improve abiotic stress tolerance in plants and the mechanism of action of the genes or enzymes involved.
Introduction
Abiotic stress, namely drought, temperature extremes (such as heat, chilling, and freezing), salinity, heavy metals, and UV radiation, can decrease plant growth and productivity by more than 50% (Khan et al., 2015; Minhas et al., 2017). Due to global warming and varying climatic conditions, abiotic stresses have become more severe and unpredictable. Furthermore, sustainable food production is also at risk due to the reduction in the availability of land for agriculture and the increasing global population (Dubey et al., 2020).
Abiotic stresses significantly limit plant productivity by disturbing cellular biochemistry and physiology via overproduction of reactive oxygen species (ROS) (Apel and Hirt, 2004). ROS are known to play a dual role in plant growth and development as both signaling molecules and harmful agents that can cause oxidative damage to plant tissues (Figure 1). Excessive ROS accumulation due to the absence of ROS redox homeostasis can have detrimental effects on plant genetic materials, such as DNA, RNA, and proteins, leading to mutations, chromosomal aberrations, and even cell death (Apel and Hirt, 2004; Nakano et al., 2006; Mittler et al., 2011; Hussain et al., 2020). ROS such as hydrogen peroxide (H2O2), superoxide radical (O2•−), hydroxyl radical (OH•) and singlet oxygen (1O2), that are produced due to excitation or incomplete reduction of molecular oxygen are harmful byproducts of normal cellular metabolism in aerobic organisms (Mittler et al., 2011).
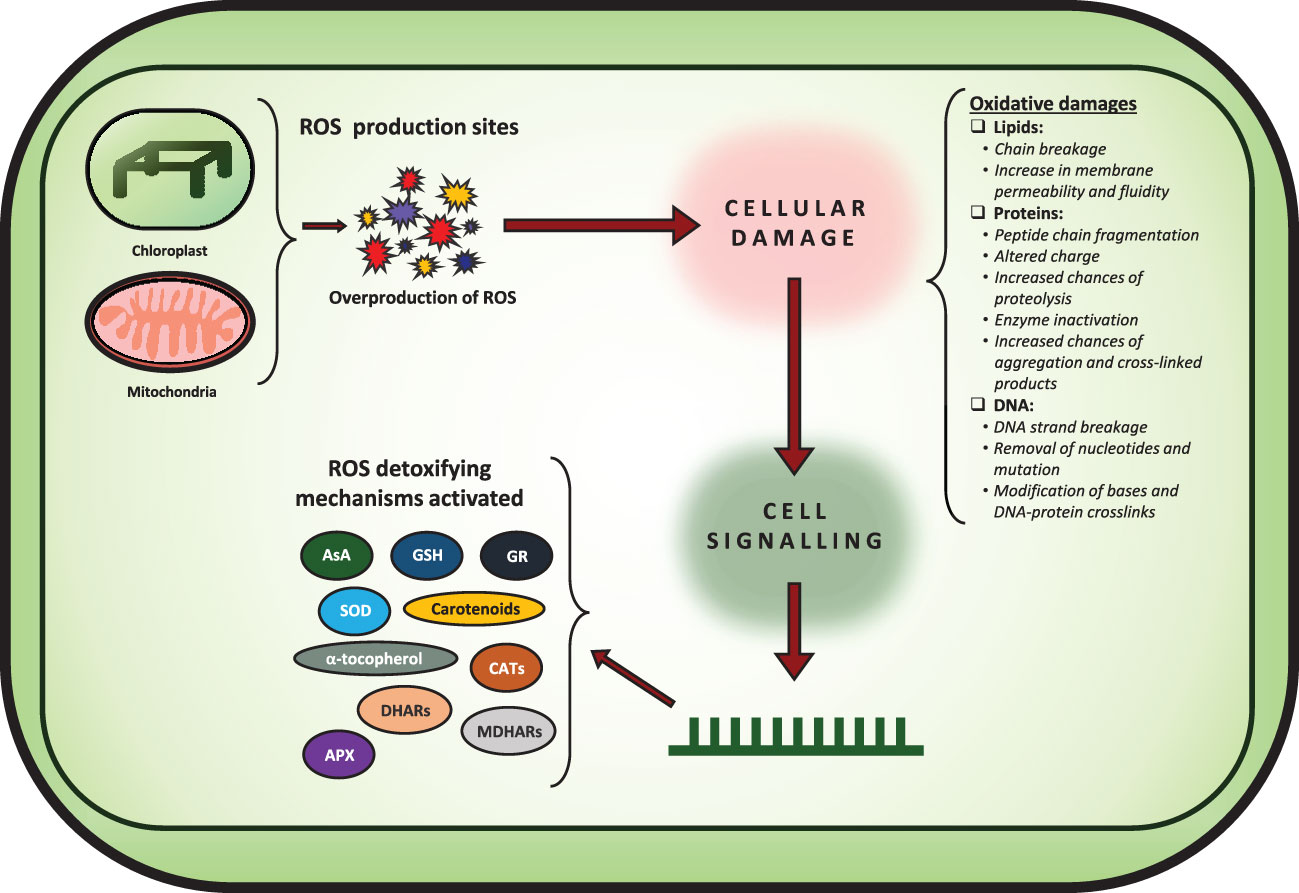
Figure 1 Figure showing how overproduction of ROS leads to various oxidative damages affecting the cellular machinery. Furthermore, the various ROS detoxifying mechanisms are shown as well.
Plants have evolved various mechanisms to cope with oxidative stress, including the production of antioxidants and the activation of stress response pathways. Several transcription factors, including WRKY and NAC, have been identified as key regulators of ROS signaling and stress response pathways (Zhu, 2016; Zhang et al., 2017; Wang et al., 2021). Studies have also shown that epigenetic modifications, such as DNA methylation and histone acetylation, can modulate the expression of stress-responsive genes and enhance plant stress tolerance (Jaleel et al., 2007; Gill and Tuteja, 2010). Plants are endowed with a variety of enzymatic and non-enzymatic antioxidants that help them scavenge excess ROS and thereby play a crucial role in ROS homeostasis. Antioxidant enzymes in plants include superoxide dismutase (SOD), ascorbate peroxidase (APX), catalase (CAT), glutathione peroxidase (GPX), monodehydroascorbate reductase (MDHAR), dehydroascorbate reductase (DHAR), glutathione reductase (GR), and glutathione S-transferase (GST) (Mittler et al., 2011). Non-enzymatic antioxidants include glutathione, ascorbate, tocopherols, carotenoids, and flavonoids. Alternatively, excess generation of ROS can be prevented via manipulation of alternative oxidase (AOX) enzymes that can prevent the leakage of electrons to oxygen (Jaleel et al., 2007). In this review, we provide an overview of ROS regulation by enzymatic and non-enzymatic antioxidants. Furthermore, we discuss the improvement of abiotic stress tolerance through ROS regulation by means of antioxidants.
Non- enzymatic antioxidants
Non-enzymatic antioxidants consist of low-molecular-weight compounds that are further categorized into water-soluble antioxidants, such as ascorbic acid and glutathione, and lipophilic antioxidants, such as tocopherols and β-carotenes. Ascorbic acid is one of the most comprehensively studied antioxidants and is present in the majority of plant cell types, organelles, and apoplasts (Gill and Tuteja, 2010; Dumanović et al., 2021). Mitochondria are the major site for ascorbate (AsA) synthesis, and ascorbate is transported through a proton-electrochemical gradient or facilitated diffusion to other cellular compartments (Gill and Tuteja, 2010). AsA is involved in the regulation of growth, metabolism, and differentiation in plants, alongside being known to scavenge free radicals and prevent oxidative damage caused by abiotic stress. In the ascorbate–glutathione (AsA-GSH) cycle, two molecules of AsA are utilized by ascorbate peroxidase (APX) to reduce hydrogen peroxide to water, generating monodehydroascorbate (MDHA) (a radical with a short lifespan). MDHA can further disproportionate into dehydroascorbate (DHA) and AsA. Also, AsA provides membrane protection by directly scavenging free radicals of oxygen and regenerates tocopherol from tocopheroxyl radical. Enhanced tolerance to ozone, salt, and polyethylene glycol was observed in tobacco when the MDHAR gene of Arabidopsis thaliana was overexpressed in tobacco (Dumanović et al., 2021). Increased AsA content was found in Picea aspertata seedlings when exposed to light and drought stress and in Cassia auriculata seedlings under UV-B stress (Eltayeb et al., 2007; Matos et al., 2022).
Tocopherols have a unique ability to quench singlet oxygen by a charge transfer mechanism. Out of all the four isomers (α, β, γ, and δ), the most biologically active is the α isomer. It is one of the most abundant antioxidants in the chloroplast membranes and is known to protect plants against photo-oxidative damage. The concentration of tocopherol increases under drought stress and high light stress, and it protects plants against oxidative damage. α-tocopherols are known to prevent the chain propagation step in lipid auto-oxidation and effectively scavenge and quench various ROS and lipid oxidation products, stabilize membranes, and regulate signal transduction (Peñuelas and Munné-Bosch, 2005; Agarwal, 2007; Pourcel et al., 2007; Shao et al., 2007; Yang et al., 2008; Bagheri et al., 2017; Kumar et al., 2020).
Glutathione, a tripeptide, occurs primarily as the reduced form (GSH), and its concentration is highest in chloroplasts (1-4 mM) in comparison to other organelles (Hajam et al., 2023). It has the ability to regenerate another powerful antioxidant, i.e., ascorbic acid via the AsA-GSH cycle (Hajam et al., 2023). Isoprenoids, such as carotenoids and tocopherols, play an important role in photoprotection as well as the response to cadmium stress (Nagajyoti et al., 2010; Nareshkumar et al., 2015). Carotenoids have the ability to delocalize unpaired electrons due to the presence of a conjugated double-bonded structure (Nareshkumar et al., 2015). They are responsible for quenching singlet oxygen without degradation and are known to chemically react with free radicals such as peroxyl (ROO•), hydroxyl (•OH), and superoxide radicals (O2•−) (Bagheri et al., 2017). Another commonly occurring antioxidant, polyphenols, is known to possess free radical scavenging activity. Phenolic compounds react with peroxyl radicals and form phenoxy radical intermediates that are rather stable and unreactive (Bagheri et al., 2017). These function as terminators of free radical chains and as chelators of redox-active metal.
Enzymatic antioxidants
Superoxide dismutase (SOD) [EC 1.15.1.1]
Overview and types
SOD (EC 1.15.1.1) is a metalloenzyme and one of the most effective components of a plant cell’s antioxidant defense system against ROS toxicity. It catalyzes the conversion of O2•− to O2 and H2O2. Initially, SOD was isolated as a green Cu-protein from bovine blood and was thought to be involved in Cu storage (Mittler, 2002). SOD is divided into three isoenzymes based on the presence of metal cofactors (Cu/Zn/Mn/Fe) at the active site (Alscher et al., 2002). All oxygen-metabolizing cells, as well as all subcellular compartments (such as chloroplasts, mitochondria, nuclei, peroxisomes, cytoplasm, and apoplasts), are assumed to contain SODs (Fink and Scandalios, 2002; Mahanty et al., 2012). Based on their localization, structure, and functions in plants, SOD can be classified into three isoenzymes, namely Cu/Zn-SOD, Mn-SOD, and Fe-SOD (Corpas et al., 2006) (Figure 2; Table 1). Cu/Zn-SOD has been found in cytosol, chloroplasts, and peroxisomes. Mn-SOD is found primarily in mitochondria, but also in peroxisomes and apoplasts (Pan et al., 2006). Fe-SOD is found primarily in chloroplasts and to a lesser extent in peroxisomes and apoplasts. A fourth group of SOD isoenzyme, Ni (II/III) at the active site (Ni-SOD), has also been discovered (Mahanty et al., 2012). Nonetheless, all SOD isoforms (Cu/Zn-SOD, Mn-SOD, Fe-SOD) are nuclear-coded and are transported to their organellar locations via NH2-terminal targeting sequences when necessary (Sharma et al., 2012). Further studies have revealed that Mn and Fe-SODs are found in both prokaryotes and eukaryotes, whereas Cu/Zn-SODs are primarily found in eukaryotes. Fe-SODs exist in homodimeric and tetrameric forms. They are resistant to KCN but not to H2O2. Similarly, Mn-SOD is homodimeric and homotetrameric, and it is resistant to both KCN and H2O2, whereas Cu/Zn-SOD is activated by both inhibitors (Gepstein and Glick, 2013). Furthermore, hormones such as abscisic acid and multifunctional signaling molecules such as melatonin regulate SOD activity via gene expression, like other antioxidant enzymes (Tuteja and Gill, 2013; Khan et al., 2020).
Response of SOD to abiotic stress
Abiotic stresses such as heat, cold, drought, salinity, and chemical contaminants are major factors limiting agricultural productivity around the world. As a result, understanding plant responses to these abiotic stresses has become a prerequisite for developing crop plants that can withstand abiotic stresses (Gepstein and Glick, 2013). Nonetheless, SODs are the first line of defense against abiotic stress because they are an important component of plant defense machinery. Due to stress, ROS and its reaction products increase, and dismutation of O2 is catalyzed by SODs into hydrogen peroxide (H2O2) and oxygen (O2). The impact of all SODs on the direct or indirect metabolism of various ROS has been confirmed (Mittler, 2006). Salinity is one of the most important abiotic stress factors that can affect the quality and yield of crops (Mittler, 2006). Although many plants have the ability to tolerate high salinity levels, most plants are susceptible to salt stress and show decreased photosynthetic activity or morphological disorders under such conditions (Sheokand et al., 2008; Szepesi et al., 2008; Zsigmond et al., 2012; Duque et al., 2013). Salinity causes an increase in ROS generation and alters the activity of ROS-scavenging enzymes. In certain plants, salt stress causes an increase in total SOD activity, while in others, no change or reduction in SOD activity was observed under abiotic stress. For example, under salt stress, increased numbers of leaves were seen in Cicer arietinum, Beta vulgaris, and Brassica juncea due to SOD activity, while a decrease in the number of leaves was observed in Vigna unguiculata (Hernandez et al., 2006; Sheokand et al., 2008; Kumar et al., 2013; Rasool et al., 2013). Furthermore, increased SOD activity under salt stress was reported in Arabidopsis thaliana and Nicotiana tobacum seedlings (Zsigmond et al., 2012; Lee et al., 2013). The significant differences in SOD activities in various plants under salt stress are evidence of its functioning at the inter-specific or intra-specific level. The effect of Cu/Zn-SOD was increased in the salt-tolerant and sensitive cultivars of Oryza sativa in response to salinity (Mishra et al., 2013). While studying the long-term effects of salt stress in two salt-tolerant lines (Kharchia65, KRL19) and two salt-sensitive lines (HD2009, HD2687) of Triticum aestivum, higher levels of SOD activity were observed in Kharchia65 when compared to HD2687 (Sairam et al., 2005). These inconsistencies show that SOD activity is influenced by a variety of factors, including the type of plant species (tolerant or sensitive), the intensity and duration of stress, and the plant organ used for the assays. Mn-SOD activity is found substantially in the cytosolic fraction. On the other hand, Kharchia65 had enhanced Mn-SOD activity in both fractions, which increases with salt stress. The activity of cytosolic Mn-SOD was very low, implying that it plays a minor role in scavenging salinity-induced O2 free-radical generation. Another isozyme, Cu/Zn-SOD, was found in all three cell compartments, with the chloroplast having the highest concentration, followed by mitochondria and cytosol. Salt stress enhanced Cu/Zn-SOD activity in both cytosolic and chloroplastic fractions in Kharchia65, but there was little or no increase in the enzyme’s activity in mitochondria. Fe-SOD was predominantly active in the chloroplastic fraction. However, the enzyme was also found to be active in the cytosolic and mitochondrial fractions. Kharchia65 showed higher Fe-SOD and Cu/Zn-SOD activity than HD2687 under both normal and salt-stress conditions. In Pisum sativum, a salinity-induced increase in chloroplastic Fe-SOD was observed (Camejo et al., 2013).
Apart from salt stress, drought stress also causes a variety of physiological changes in plants, including a decrease in CO2 fixation due to abscisic acid-mediated stomatal closure, which leads to a reduction in photosynthetic rate and/or morphological abnormalities, mostly lower organ growth (Anjum et al., 2011). Furthermore, an increase in ROS production is associated with lipid peroxidation and alterations in enzymatic and non-enzymatic antioxidants (Csiszar et al., 2007; Tari et al., 2008; Dinakar et al., 2012). It has been found that total SOD activity appears to increase in the leaves of Olea europaea and the shoots of Oryza sativa cultivars when exposed to water stress (Sharma and Dubey, 2005; Sen and Alikamanoglu, 2013). However, an increase in total SOD activity was followed by a decrease in the number of leaves of Gossypium sp. and two grass species (Festuca arundinacea and Poa pratensis). On the flip side, a significant reduction of SOD activity was noticed for the pea plant, under drought stress (Deeba et al., 2012; Doupis et al., 2013). SOD activity enhanced or remained constant in Triticum aestivum during the early stages of drought but declined with chronic water stress (Deeba et al., 2012). Studies suggest that photosystem II can be protected by SOD from reactive O2 induced by oxidative and water stress (Deeba et al., 2012). In a recent study, the Tibetan wild Hordeum vulgare genotypes XZ16 and XZ5 were found to have considerably elevated SOD activity throughout the anthesis period when subjected to drought stress (Ahmed et al., 2013).
Cold stress is another major abiotic stress that can cause functional abnormalities in chloroplasts or mitochondria, resulting in ROS overproduction (Latef, 2021). Earlier research demonstrated that plants produce a lot of H2O2 during cold stress, and higher antioxidant enzyme activity tends to correlate with higher tolerance (Huang and Guo, 2005a). Studies in tolerant rice, barley, and tobacco cultivar roots and shoots revealed a differential amount of SOD activity under cold stress (Huang and Guo, 2005a; Xu et al., 2010; Mutlu et al., 2013). In sensitive as well as tolerant cultivars of tobacco the roots exhibited a higher SOD and catalase activity as compared to peroxidase activity (Xu et al., 2010). While tobacco shoots exhibited a higher peroxidase activity in comparison to SOD and catalase activity (Xu et al., 2010). In paraquat-treated Nicotiana plumbaginifolia, increased mRNA levels for mitochondrial Mn-SOD, chloroplastic Fe-SOD, and cytosolic Cu/Zn-SOD were observed in the presence of light. However, only cytosolic Cu/Zn-SOD expression was observed in the dark (Mylona et al., 2007). Further, convincing evidence was found for the stimulation of genes and enzyme activity in Zea mays embryos in response to paraquat. Benzyl viologen-produced O2 and O2• was found to increase the expression of mitochondrial Mn-SOD and cytoplasmic Cu/Zn-SOD genes (Mylona et al., 2007). O3 (ozone) is a contaminant in the atmosphere that breaks down in the apoplast to produce primarily O2• and H2O2. Acute or chronic exposure of Zea mays seedlings resulted in increased transcript levels of mitochondrial Mn-SOD and cytosolic Cu/Zn-SODs in the leaves (Rossa et al., 2002). On the other hand, the transcript level of chloroplastic Cu/Zn-SOD was shown to be down-regulated. The blue light wavelength has been shown to induce SOD activity more effectively in Gracilariopsis tenuifrons than other wavelengths (Rossa et al., 2002). UV B (280-350 nm) irradiation increased SOD activity in Cassia auriculata seedlings (Agarwal, 2007). Expression of Mn-SOD enzyme was also increased in Nicotiana tabacum leaves when exposed to high light intensity. However, increased total SOD activity was observed in Hordeum vulgare catalase-deficient mutants RPr 79/4 after the plants were exposed to high intensities of light (Azevedo et al., 1998; Dat et al., 2003).
Genetic engineering of plant SOD
The activation of SOD in response to various environmental conditions, as discussed in the previous section, suggests that it is a significant component of the plant’s defense system, and therefore, its genetic modification could lead to the development of stress-tolerant phenotypes. It was observed that Oryza sativa showed better resistance to methylmercury and drought stress when the Pisum sativum Mn-SOD gene was expressed in the chloroplasts (Wang et al., 2005). In another instance, overexpression of the Cu/Zn-SOD gene in Nicotiana tabacum plants was done to improve oxidative stress resistance, while tolerance to methyl viologen and pure cercosporin was achieved in sugar beet by transferring a Lycopersicon esculentum SOD gene (Tertivanidis et al., 2004; Wang et al., 2005). SOD genes have been shown to help plants eliminate ROS more efficiently under methyl viologen exposure or environmental stresses such as cold, ozone, water deficit, and salt stress (Wang et al., 2005). Nonetheless, there are several transgenic plants with SOD genes from different plants that did not show any improvement in stress tolerance (Tertivanidis et al., 2004; Sunkar et al., 2006). The differences in SOD isoenzymes, as well as the complexity of the ROS detoxification system, have been proposed as key variables for these instances (Kwon et al., 2002; Jia et al., 2009). When Mn-SOD was overexpressed in Arabidopsis, Mn-SOD, Cu/Zn-SOD, and Fe-SOD activity was higher in transgenic plants than wild-type plants, and the transgenic plants grew well and produced fewer lipid peroxidation products when treated with 150 mM NaCl (Mylona and Polidoros, 2010). The transcriptome of knockdown Arabidopsis plants with suppressed expression of chloroplastic Cu/Zn-SOD (CSD2) revealed the appearance of induced chloroplast and nuclear-encoded genes as a result of O2 buildup under optimal conditions (Mylona and Polidoros, 2010). Mn-SOD gene from halophilic archaeon was extracted and transferred into Oryza sativa by agrobacterium-mediated transformation to explore new gene resources for increasing Oryza sativa tolerance to salt stress (Wang et al., 2004). The transformants (L1 and L2) showed some MnSOD expression and increased overall SOD activity, resulting in improved ROS removal efficiency under salt stress. The amounts of oxygen and hydrogen peroxide (H2O2) were dramatically reduced; they also had better levels of photosynthesis and lower levels of relative ion leakage and malondialdehyde (MDA) concentration than wild-type plants (Wang et al., 2004). In contrast to the reports discussed above, overexpression of cytosolic or chloroplastic SOD in some transgenics only provided moderate or minimal tolerance, owing to the type of overexpressed SOD and its subcellular localization (Wang et al., 2004). Cu/Zn-SOD and FeSOD inactivation by H2O2, their reaction end product, and the competing resistance of Mn-SOD to H2O2 may be relevant to their specific effects. In this scenario, antisense technology is now feasible and should be used in conjunction with any overexpression research.
Ascorbate peroxidase (APX) [EC 1.11.1.11]
Overview and types
Ascorbate peroxidase is a heme-containing peroxidase that catalyzes the oxidation of a wide spectrum of organic compounds in the presence of H2O2. APX is found in higher plants, chlorophytes, red algae, and members of the protist kingdom, and plays a crucial role in growth regulation (Wang et al., 2004; Chen et al., 2013). The peroxidase database contains APX and other peroxidase sequences from all kingdoms of life, as well as a set of bioinformatics tools for evaluating peroxidase sequences. Genomic and cDNA APX sequences have been discovered from a wide range of plants, demonstrating that APX is found throughout the vegetal domain. In these organisms, small gene families encode this enzyme (Oliva et al., 2009; Chen et al., 2013). The different isoforms of APX are categorized based on subcellular localization in the cell (Figure 3). Membrane-bound isoforms are present in microbodies (including peroxisome and glyoxysome) and chloroplast thylakoids, while soluble isoforms are found in the cytosol, mitochondria, and chloroplast stroma. The subcellular localization of the isoenzyme is determined by the presence of organelle-specific targeting peptides and transmembrane domains in the N and C-terminal regions of the protein (Teixeira et al., 2004; Passardi et al., 2007).
Furthermore, the plant chloroplastic APX isoenzyme-encoding genes are divided into two groups in plants. The first group consists of single genes that encode two isoenzymes. Genes from spinach (S. oleracea), tobacco (N. tabacum), pumpkin (Cucurbita sp), and ice plant (Mesembryanthemum crystallinum) are included in this group. Individual genes code for distinct isoenzymes that are separately regulated in the second category. Arabidopsis, rice, and tomato genes are included in this group. In spinach, the mechanism of alternative splicing in chlAPX was investigated, and the results revealed that alternative splicing is critical for modulating the expression of stromal (sAPX) and thylakoid (tAPX) isoenzymes, and this regulation happens in a tissue-dependent manner. In certain plant species, ascorbate peroxidases have been partially identified. The APX family in spinach is composed of genes that code for one cytosolic, two chloroplastic (sAPX and tAPX membrane) isoenzymes, one microbody membrane-targeted isoenzyme, and an unknown putative cytosol-soluble isoenzyme (Teixeira et al., 2006). Four cDNAs belonging to potential cytosolic, peroxisomal, and chloroplastic (thylakoid and stromal) APX isoforms were identified and studied in Cowpea (D’Arcy-Lameta et al., 2006). Six APX-coding loci were discovered in Eucalyptus grandis, with prediction tools indicating their subcellular localization. Three out of six isoforms were predicted to be cytosolic, one as a putative peroxisomal protein, and two as chloroplast-associated proteins (Teixeira et al., 2005). Another study in tomato revealed seven members where three were cytosolic, two peroxisomal, and two chloroplastic members (Najami et al., 2008). Two chloroplastic, one thylakoid-bound, and one membrane-bound, whose product is targeted to both chloroplast stroma and mitochondria, were identified in the model plant Arabidopsis thaliana, with the intracellular localization of an additional member still being unknown (Najami et al., 2008). Two chloroplastic, one thylakoid-bound, and one membrane-bound APX, whose product is targeted to both chloroplast stroma and mitochondria, were identified in the model plant Arabidopsis thaliana, while the intracellular localization of an additional member is still unknown (Najami et al., 2008). The APX gene family of rice has a total of eight members, with two members each in cytosol, peroxisome, chloroplast, and mitochondria. In rice, a novel protein called APX-R (Ascorbate peroxidase-related) has recently been discovered to be functionally connected with APX (Lazzarotto et al., 2011). Further analysis revealed that the Apx-R gene corresponds to a new class of heme peroxidases (Lazzarotto et al., 2011).
In the absence of AsA, APX isoenzymes are labile, and thus, a high quantity of endogenous AsA is required for the antioxidant system to effectively protect plants from oxidative damage (Maruta et al., 2010). The APX activity is quickly lost under particular conditions when the concentration of AsA is less than 20 μM, making chlAPX the least stable isoform. Both cAPX and mAPX have half-inactivation times of one hour or more, whereas mAPX and chlAPX have half-inactivation times of less than 30 seconds (Anjum et al., 2016).
Response of APX under different abiotic stresses
Drought stress, salt stress, high levels of light, high and low temperatures, pathogen attacks, H2O2, and abscisic acid all affect the expression of APX-producing genes (Teixeira et al., 2004; Passardi et al., 2007; Anjum et al., 2016). Furthermore, the transcriptional expression of APX genes varies depending on tissue and developmental stage (Teixeira et al., 2005). By restricting the amount of water available to the plant, salinity stress causes ion imbalances and physiological drought-like conditions. In such situations, APX provides varied levels of salt tolerance to affected plants. Salinity-induced oxidative damage is seen in cAPX mutants, but constitutive overexpression lines demonstrate increased resistance to 100 mM NaCl stress (Diaz-Vivancos et al., 2013). Tomato plants that overexpress pea cAPX are found to be more resistant to salinity stress (Wang et al., 2005). In transgenic Arabidopsis plants, overexpression of OsAPX2 exhibits higher tolerance to salt stress than OsAPX1. However, it is possible that the observed differences in tolerance are attributable to the positional influence of distinct transgenic lines. In transgenic tobacco, a cAPX from Arabidopsis displayed enhanced salt, drought, and PEG tolerance. Furthermore, in sensitive plants, salt stress causes lipid peroxidation and membrane damage, as well as low levels of antioxidant enzymes. Transgenic tobacco BY-2 cell lines with 50 and 75 percent decreased cAPX activity revealed higher ROS buildup. The study found that ascorbate peroxidase gene expression during stress resulted in salt and heat tolerance with no significant changes in levels of other ROS scavenging enzymes (Ishikawa et al., 2005; Wang et al., 2005; Nakano et al., 2006; Lu et al., 2007; Berwal et al., 2021).
Pea chloroplast APXs responded differently in saline conditions, with sAPX gradually increasing and tAPX gradually dropping, but a tAPX from Solanum lycopersicum introduced in tobacco gave enhanced resistance to salt and osmotic stress. Under salt stress, chlAPX activity increases, which protects against ROS generated in mitochondria and/or peroxisomes. Transgenic tobacco accumulating higher ascorbate was used to induce salt stress tolerance. Drought and salt conditions increased the expression of the APX gene in French bean seedlings (Eltelib et al., 2012; Nageshbabu and Jyothi, 2013). In Arabidopsis, overexpression of an APX from Puccinellia tenuiflora, a salinity-tolerant wild grass, boosted tolerance to 175 mM NaCl while also protecting against lipid peroxidation. Under salinity conditions, transcripts for mAPX from Hordeum vulgare, while a Populus peroxisomal APX in tobacco, conferred salt, drought, and MV stress resistance as well as larger roots (Li et al., 2009; Eltelib et al., 2012). Furthermore, APX mutants can upregulate other peroxidases to compensate for the loss of APX and give stress tolerance. This is validated by the expression of rice GPX in rice APX 1/2 mutants, as well as the upregulation of other enzymes (CAT and GP) in Arabidopsis APX3 knockout mutants that showed no indications of stress. When salicylic acid is administered topically, it stimulates APX and GR activity, resulting in increased salt tolerance in mung bean (Narendra et al., 2006; Bonifacio et al., 2011; Nazar et al., 2011; Guan et al., 2015; Husen et al., 2018). The response of all antioxidant enzymes to salt stress in Brazilian indica rice was studied at two developmental phases, and it was discovered that cAPX expression was up-regulated in 11-day-old seedlings but not in 6-week-old plants (Guan et al., 2015). This stress causes the production of ROS. The response of APX isozymes to this circumstance at different phases of plant growth is regulated. Under salinity stress, the normal salinity-resistant rice leaf basal region showed an increase in CAT and APX transcripts, whereas APX8 levels were slightly reduced. Under saline conditions, the expression of OsAPX2 did not change (Yamane et al., 2010). Another study found a similar drop in APX8 responsiveness when the other isoforms, APX2 and APX7, were strongly expressed during salt stress. In a different experiment, OsAPX8 demonstrated high expression in rice roots over a wide range of salt concentrations, from 150 to 300 mM, but OsAPX7 transcripts dropped dramatically at 300 mM. Age, cultivar, plant sections, and physiological conditions of plant growth all contribute to variances in APX gene expression (Teixeira et al., 2004; Hong et al., 2007; Passardi et al., 2007). Sweet potato plants with various levels of sensitivity to salt stress accumulated APX transcripts differently, with larger quantities in tolerant genotypes. APX plays a key function in plant drought resistance and recovery. Overexpressed P5CS gene in transgenic soybean and tobacco under drought stress elevate APX transcripts. In Prunus sp., APX and other Asc-GSH pathway enzymes were up-regulated during drought treatment and reduced throughout the recovery period (Sofo et al., 2005b; Teixeira et al., 2006; Zarei et al., 2012; Cruz et al., 2013). Studies have reported that glycine betaine (GB) increases APX under drought (Sofo et al., 2005b; Teixeira et al., 2006; Zarei et al., 2012; Cruz et al., 2013). Overexpression of cAPX (APX1) reduces drought symptoms, and transgenic tobacco plants outperformed non-transgenic plants. The relevance of this isoform in plant growth and development was also demonstrated by loss of function APX2 mutants, which were more vulnerable to drought than over-expression lines. Drought resistance was improved in over-expression lines of an mAPX from Salicornia brachiata relative to control plants (Zhang et al., 2013; Singh et al., 2014). cAPX is found to be important in drought stress tolerance in tobacco, with a primary benefit being membrane protection (Faize et al., 2011). Even under non-stressed conditions, APX activity is observed to be higher in tolerant cowpea plants. The sensitive cultivar up-regulates cAPX and mAPX in response to stress, whereas the tolerant cultivar up-regulates chlAPX (D’Arcy-Lameta et al., 2006). Various wheat genotypes have different levels of APX expression when there is a water crisis. cAPX1 was up-regulated in both genotypes, sAPX2 only in sensitive, and tAPX and cAPX2 only in tolerant cultivars (Secenji et al., 2009). After 15 days of drought stress in rice, the tAPX gene was down-regulated, while numerous other isoforms were upregulated. However, certain microsomal isoforms were only slightly or not at all altered (Rosa et al., 2010). This indicates that APXs are expressed differently in different species and under different conditions.
The temperatures that are either too low or too high have an adverse impact on plant physiology. Cold stress induces APX expression in tolerant maize lines, but not in sensitive ones (Caverzan et al., 2012). When compared to heat stress, low temperatures increase cAPX expression in potato tubers, implying that it plays a role in cold adaptation (Caverzan et al., 2012). Tobacco plants with a higher level of tAPX were more resistant to freezing and light stress, whereas Arabidopsis plants with a lower level of tAPX were more resistant to heat stress (Miller et al., 2007; Wang et al., 2016). Rice plants with homologous overexpression of a cAPX were tolerant to colder temperatures at the booting stage than wild-type plants, owing to enhanced APX activity in spikelets (Sato et al., 2011). SOD and APX gene expression in potato chloroplasts was induced using an inducible promoter SWPA2 that works under oxidative stress. With a substantial difference from the control, the plants obtained were tolerant to higher temperatures and methyl viologen (MV) stressors (Tang et al., 2006). In a similar experiment with sweet potatoes, tolerance to cold and MV stressors was observed (Lim et al., 2007). Transgenic plants with tomato tAPX expressed in tobacco were more resistant to both temperature and light stressors, and their photosynthetic efficiency was higher than non-transformed plants (Sun et al., 2010). High temperature increases cAPX in sweet potato leaves, but cAPX, mAPX, and sAPX were all up-regulated in cucumber after an initial decline (Park et al., 2004; Song et al., 2005). A cAPX has been reported to decrease quickly after heat shock treatment, negating its positive role in this stress, whereas some studies claim that APX2 is increased under heat circumstances (Gao et al., 2010). In Arabidopsis cells, APX1 is known to be active largely in response to heat and drought stress (Koussevitzky et al., 2008). In Arabidopsis, a mAPX from barley was overexpressed to display heat stress tolerance (Smeets et al., 2008). As a result, different APX isoforms and antioxidative mechanisms at multiple subcellular sites can be used to breed plants that can withstand environmental stress.
Heavy metal ion pollution in the soil is a major problem that reduces crop productivity. Under cadmium and arsenic stress, APX expression was induced in the leaves of Arabidopsis thaliana, Solanum nigrum, and Brassica juncea, but it was reduced in the leaves of Brassica napus (Smeets et al., 2008; Khan et al., 2009; Markovska et al., 2009; Nouairi et al., 2009; Pinto et al., 2009; Ansari et al., 2015). Copper stress increased APX expression in leaves of Elsholtzia splendens, however it was variable in Withania somnifera depending on metal ion concentrations (Peng et al., 2006; Khatun et al., 2008). The expression of APX isoforms in Nicotiana tabacum and Typha angustifolia leaves was observed to remain constant with varying levels of cadmium stress, while chromium and lead stresses did not cause any changes in APX expression in Typha leaves (Bah et al., 2011). Cadmium stress caused APX expression to vary in Zea mays (Ekmekçi et al., 2008). Low concentrations of cadmium stimulated APX activity in cells of coffee plant, but higher concentrations had no effect after 24 hours, while nickel enhanced APX activity at two extreme concentrations (Gomes-Junior et al., 2006). In rice, aluminium exposure causes practically all APX isoforms to become active. Double mutants of cAPX1/2 had a higher tolerance to high concentrations of aluminium (Sharma and Dubey, 2007; Rosa et al., 2010). This heavy metal enhances cAPX activity in pea at higher concentrations for longer periods of time, whereas it decreases and becomes constant beyond it (Panda and Matsumoto, 2010). De-rooted bean plants with inadequate cAPX were susceptible to iron, as were tobacco plants with deficient cAPX (Caverzan et al., 2012). Copper and cadmium increased APX activity in transgenic tall fescue plants compared to control, but arsenic decreased it in both transgenic and control plants (Lee et al., 2007). In Eichhornia crassipes seedlings, lead stress boosted APX activity (Lee et al., 2007). Cadmium chloride boosted APX activity in salt tolerant and sensitive rice cultivars, with the former having a higher activity (Roychoudhury and Ghosh, 2013; Malar et al., 2014). A similar increase in APX activity was seen in Vigna radiata (Roychoudhury and Ghosh, 2013). Salt and lead stress doubled on Vigna radiata seedlings resulted in an increase in APX activity (Siddiqui, 2013). As a result, several scientific publications have shown that APXs play a significant role in protecting plants from heavy metal stress in soil (Siddiqui, 2013).
Catalases (CAT) [EC 1.11.1.6]
Overview
Catalases are tetrameric heme-containing enzymes that convert hydrogen peroxide to water and oxygen and are mostly found in peroxisomes (Srivalli et al., 2003). Catalase isozyme forms are found in many plants, including two in castor bean and six in Arabidopsis, and they can directly dismutate H2O2 or oxidize substrates such as methanol, ethanol, formaldehyde, and formic acid (Ben-Amor et al., 2005).
Plant catalases are divided into three groups based on their structures: class 1 catalases are found in photosynthetic tissues and are involved in the removal of H2O2 produced during photorespiration; class 2 catalases are found in vascular tissues and may play a role in lignification, though their exact biological role is unknown; and class 3 catalases are found in seeds and young plants, and their activity is linked to the removal of excess H2O2 produced during fatty acid degradation in the glyoxylate cycle in glyoxisomes (Ben-Amor et al., 2005). Catalases are the primary scavenging enzymes that may directly dismutate H2O2 and are required for ROS detoxification during stress (Ben-Amor et al., 2005). This is also related to the fact that during stress, peroxisomes proliferate, possibly aiding in the scavenging of H2O2 that diffuses from the cytosol (Ben-Amor et al., 2005). Increased catalase activity is thought to be an adaptive characteristic that could aid in overcoming tissue metabolic damage by lowering harmful levels of H2O2 (Mhamdi et al., 2010). In these organelles, a 200mM NaCl concentration resulted in a decrease in catalase activity (Srivalli et al., 2003). Increased catalytic activity in transgenic tobacco with sense cDNA of cotton catalase was shown to reduce photorespiratory loss, but antisense constructions reduced catalase specific activity, resulting in a commensurate increase in the CO2 compensation point. In alfalfa nodule, tea, cotton, and tobacco, abiotic stress causes upregulation of the genes responsible for catalase expression (Sekmen et al., 2007; Upadhyaya et al., 2008; Vital et al., 2008; Mhamdi et al., 2010). Catalases of class II have mostly been examined in relation to disease progression and resistance. It has been discovered that they are a target for SA (salicylic acid), and transgenic potato plants expressing the tobacco Cat2Nt gene could result in constitutive expression of the endogenous potato Cat2St gene and increased resistance to Phytophthora infestans (Vital et al., 2008). In dry and arid areas, two of the most common and frequent abiotic stresses are drought and salinity. Vegetation experiencing salt and drought stresses has evolved a range of physiological mechanisms to cope with harsh climatic circumstances (Zhang et al., 2008; Deeba et al., 2012). Abiotic stresses in semi-arid regions result in a loss of plant growth and productivity, which leads to several developmental, physiological, cellular, and molecular responses (Grover et al., 2011; Pinheiro and Chaves, 2011; Sobhanian et al., 2011). The majority of these responses are caused by photon intensity beyond the absorption capacity of stressed plants (Figure 3). Photorespiration is known to allow oxygenic photosynthesis by scavenging its most poisonous by-product, 2-phosphoglycolate, but it also causes substantial losses of freshly assimilated CO2 from most land plants (Rahman et al., 2023). Many studies have focused on the importance of the CAT catalysis pathway under drought and salt stress because of the critical involvement of CAT in photorespiration. Indeed, the persistence of CAT activity in drought-stressed plants’ leaves is likely responsible for the elimination of photorespiratory H2O2 generated when plants are subjected to higher levels of water deficit and salinity stresses. Photorespiration acts as an energy sink in these conditions, limiting photoinhibition and over-reduction of the photosynthetic electron transport chain (Bauwe et al., 2012). On this basis, photorespiration and the CAT pathway are no longer regarded as wasteful activities, but rather as critical and accessory components of photosynthesis and aspects of stress responses in green tissues for preventing ROS accumulation (De Pinto et al., 2013; Rahman et al., 2023). Drought stress and salt predispose the photosynthetic system to photoinhibition, leading to light-dependent inactivation of the principal photochemistry associated with photosystem II that often persists after rewatering (Deeba et al., 2012).
Indeed, decreased CO2 transport to the chloroplast and metabolic restrictions influence photosynthesis, which is one of the primary activities affected by water deficiencies and high salt concentrations (Grover et al., 2011; Pinheiro and Chaves, 2011). Due to the concurrent or even earlier suppression of growth, total plant carbon uptake is further reduced. Water deficiency, either directly or indirectly resulting in lower growth, has a significant impact on leaf carbohydrate status and hormonal balance. Increased levels of reactive oxygen species (ROS) such as superoxide anion (O2-), hydrogen peroxide (H2O2), and hydroxyl radicals are commonly related to plant adaptation to drought and salinity. ROS are by-products of aerobic metabolism, and their generation is boosted by the disturbance of the electron transport system and oxidizing metabolic processes in chloroplasts, mitochondria, and microbodies during stressful situations (Grover et al., 2011; Voss et al., 2013; Ramakrishna and Gill, 2018; Rajput et al., 2021). ROS are effectively eliminated by non-enzymatic and enzymatic antioxidants in non-stressful conditions, but during drought and saline conditions, ROS production surpasses the ability of antioxidative systems to remove them, resulting in oxidative stress (Vanderauwera et al., 2012; Nawaz et al., 2021). Catalase (CAT) isoforms are iron porphyrin enzymes that act as an efficient ROS scavenging system to protect cells from the oxidative damage caused by these two stressors (Mittler et al., 2011). Based on previous research, an increase in CAT activity is often connected to the degree of dryness that plants experience (Grover et al., 2011; Mittler et al., 2011). The root length increases gradually in Panicum sumatrense under drought stress at all growth stages, whereas the chlorophyll pigments and plant height decrease (Vanderauwera et al., 2012). According to the researchers, compatible solutes such as proline, glycine betaine, and free amino acid increased in all drought treatments (Ajithkumar and Panneerselvam, 2014; Nawaz and Wang, 2020). Furthermore, stress treatment increased the activity of antioxidant enzymes such as superoxide dismutase (SOD), catalase, and peroxidases, enabling this species to exhibit strong drought-tolerance characteristics. Leaf CO2 absorption rate and carboxylation efficiency characteristics decreased as the water deficit increased in another drought-tolerant species (Jatropha curcas). Leaf H2O2 level and lipid peroxidation were negatively and strongly linked with CAT activity in this species, indicating that drought-induced suppression of this enzyme could have a negative impact. Differences in antioxidant responses to drought in C3 and C4 plants are few, but they may be essential in understanding the metabolic antioxidant patterns of these two plant groups. Relative shoot growth rate, relative water content and osmotic potential, H2O2 content and nicotinamide adenine dinucleotide phosphate (NADPH) oxidase activity, CAT activity, CAT1 mRNA level, and lipid peroxidation were studied in Cleome spinosa (C3) and Cleome gynandra (C4) seedlings. Seedlings grown under control conditions consistently had higher antioxidant enzymes (excluding SOD) in Cleoma spinosa than in Cleoma gynandra (Ajithkumar and Panneerselvam, 2014). CAT activity was linked with CAT1 gene expression in Cleoma spinosa, but not with CAT1 gene expression in Cleoma gynandra for 10 days. Drought stress increased the levels and activity of the CAT enzyme in both species. The findings revealed that the antioxidant defense system in Cleoma spinosa was unable to limit the increased ROS generation under stress. The antioxidant system in Cleoma gynandra, on the other hand, was able to cope with ROS generation under drought stress, despite its induction being lower than in Cleoma spinosa. Ford et al. investigated the quantitative changes in protein abundance of three Australian bread wheat cultivars (Triticum aestivum L.) in response to drought stress using a series of multiplexed experiments (Uzilday et al., 2012). The three cultivars, namely Kukri (drought-intolerant), Excalibur (drought-tolerant), and RAC875 (drought-tolerant), were produced in the glasshouse with cyclic drought treatment that replicated field conditions. The proteome modifications in the three cultivars at different times during the water shortage period represented their physiological responses to drought. An increase in CAT and SOD isoforms, as well as a decrease in proteins involved in photosynthesis and the Calvin cycle, were seen in all three cultivars, indicating an increase in oxidative stress metabolism and ROS scavenging capacity, as well as ROS avoidance.
Using a transgenic wheat line, researchers evaluated the response of photosynthesis to drought, heat stress, and their combination in the same species (Ford et al., 2011). According to the study, all stresses reduced photosynthesis, but their combination amplified the negative impacts. For instance, drought stress was found to reduce the transpiration rate, stomatal conductance, and intercellular CO2 concentration. On the other hand, heat stress boosted these photosynthetic characteristics, but it also decreased antioxidant enzyme activity, and hence, the antioxidative defense system. Given the difficulty of examining biochemical and molecular responses in the field, where a variety of factors other than dryness play a crucial role, scientific work on CAT in tree species is uncommon. Olive plants were found to up-regulate the enzymatic antioxidant system under water deficient conditions (Wang et al., 2010). This reaction protects the cellular machinery and reduces ROS-induced cellular damage. In fact, CAT activity increased significantly in plant leaves subjected to drought stress. The significant increase in CAT activity found in leaves may protect chloroplasts, which are the principal generators and targets of ROS action and present persistent electron fluxes under stress conditions (Sofo et al., 2005a). Under drought conditions, the efficiency of autochthonous plant growth-promoting rhizobacteria (Bacillus megaterium (Bm), Enterobacter sp., Bacillus thuringiensis, and Bacillus spp.) was investigated in Lavandula dentata and Salvia officinalis (Foyer and Shigeoka, 2011). In these two plant species, each bacterium used various ways to ameliorate water constraint and drought stress, including CAT up-regulation. It was found that plant characteristics, such as a low shoot/root ratio and a high stomatal conductance, are essential determinants limiting bacterial effectiveness in increasing nutritional, physiological, and metabolic plant activities (Armada et al., 2014). Salinity in agricultural land is a serious concern worldwide, which puts severe constraints on crop growth and productivity in many places (Zhang et al., 2008; Tanou et al., 2012; Armada et al., 2014). High salinity causes water deficit and ion toxicity in many plant species, and their sensitivity to salt stress varies. Compatible solutes, such as proline, trehalose, and glycine betaine, are deposited at millimolar concentrations in transgenic plants under salt stress, acting as osmoprotectors in physiological responses and allowing the plants to better withstand soil salinity (Chen and Murata, 2011; Vanderauwera et al., 2011). Low levels of GB, administered exogenously, or created by transgenes for the production of suitable solutes, can also trigger the expression of stress-responsive genes, such as those responsible for scavenging reactive oxygen species (Vanderauwera et al., 2011). Furthermore, significant efforts have been made to investigate how genes react to salt stress in many organisms. The effects of NaCl on H2O2 content and CAT activity were investigated in various plants, including a single-celled alga (Chlorella sp.), an aquatic macrophyte (Najas graminea), and a mangrove plant (Suaeda maritima), which have a high tolerance to NaCl. When exposed to high levels of NaCl, all the examined plants produced considerable amounts of H2O2, and CAT activity rose dramatically in response to the NaCl treatment (Nounjan et al., 2012). Interestingly, the same investigators discovered that cultivating the plants in a high-salinity environment led to the generation of novel CAT isoforms. A gene encoding a small GTPase (MfARL1) from a subtracted cDNA library in Medicago falcate was identified to better understand the role of certain essential genes in the response to salt stress (Mallik et al., 2011).
Under salt stress, transgenic seedlings that constitutively express MfARL1 showed a higher survival rate. Salt stress significantly reduced chlorophyll concentration in wild-type plants, but not in transgenic plants. During these saline conditions, transgenic plants accumulated less H2O2 and showed less oxidative damage than their wild-type counterparts, which can be attributed to higher CAT activity. Peroxisomal CAT activity was found to be higher in tomato leaves and roots treated with various degrees of salt stress compared to controls, although CAT activity in pure leaf peroxisomes did not increase in response to salinity in other species such as peas (Wang et al., 2013). AtWNK8, mostly expressed in the primary root, hypocotyl, stamen, and pistil, appears to play a crucial role in salt and osmotic stress tolerance (Yang and Guo, 2018). Indeed, mutants overexpressing the WNK8 gene are more resistant to salt and osmotic stressors than the wild type (Yang and Guo, 2018). CAT activity in WNK8 mutants is higher than in wild-type plants under NaCl and sorbitol stress. This study provides evidence that the improved resistance of WNK8 mutants to salt stress is due to increased endogenous activities of CAT and GPX in association with increased proline synthesis and accumulation. Some plant pretreatments have been identified as effective ways to stimulate plant defenses against salt stress. For instance, the effects of two exogenous osmoprotectants (proline and trehalose) on a salt-sensitive rice variety reveal that salt stress caused growth reduction, an increase in the Na+/K+ ratio, an increase in proline level, and up-regulation of proline synthesis genes (pyrroline-5-carboxylate synthetase, P5CS) (Chen and Murata, 2011). Exogenous osmoprotectants did not appear to ameliorate growth inhibition during salt stress, but they appeared to have a significant favorable effect during the recovery period, with a larger percentage of growth recovery. The scientists discovered that an increase in CAT activity was linked to a considerable decrease in H2O2, especially in proline-treated plants. Administering proline to tree species (different wild almond species) can reduce the negative impacts of abiotic stresses like salinity, allowing leaves to better withstand oxidative stress by functioning as an effective H2O2 scavenger (Zhang et al., 2013). Furthermore, salt stress has been shown to cause considerable alterations in CAT activity in a variety of wild almond species (Sorkheh et al., 2012a). One study investigated the effects of H2O2 leaf spraying pretreatment on plant growth, and it was found that spraying H2O2 boosted antioxidant enzyme activity, with CAT being the most sensitive (Sorkheh et al., 2012b). Considering the protective effect of CAT, increased CAT activity appears to be linked to gene expression regulation, and decreased oxidative damage was identified in plants with higher CAT activity.
Glutathione reductase (GR) [EC 1.8.1.7]
Overview
Glutathione Reductase (GR or GSR) is a flavoprotein oxidoreductase that helps catalyze the reduction of glutathione disulfide (GSSG) to its reduced sulfhydryl form (GSH) using NADPH as a reductant. The reduced GSH formed is then utilized for the regeneration of ascorbic acid (AsA) using monodehydroascorbate (MDHA) and dehydroascorbic acid (DHA), thereby converting GSH to GSSG (Figure 4). GR has been shown to play a pivotal role in the plant defense against reactive oxygen metabolites generated by various abiotic stress conditions to which the plant is exposed (Gondim et al., 2012; Das and Roychoudhury, 2014; Aftab and Hakeem, 2022). Studies have described glutathione reductase as “a boon in disguise for abiotic stress defense operations”. GR efficiently maintains a relatively high cellular GSH/GSSG ratio by catalyzing the formation of a disulfide bond in glutathione disulfide (Gondim et al., 2012; Aftab and Hakeem, 2022). This enzyme is predominantly localized in the stroma of the chloroplast, but its isomers can also be found in mitochondria, cytosol, and peroxisomes (Gill et al., 2013). The enzyme is a homodimer of flavin adenine dinucleotide (FAD) having a molecular mass ranging from 100 to 150 kDa (Figure 5). An active site is located between the FAD binding domain and NADPH binding domain where the GSSG is bound (Ahmad et al., 2010). There is an additional interface region on each of the monomers of FAD that not only helps the GSSG to be bound between the subunits but also brings the FAD domains of each subunit in close proximity with the opposite catalytic site (Ahmad et al., 2010). It has been observed that in the absence of thiols, glutathione reductase has a tendency to form tetramers. However, GSH formed helps to maintain GR in its homodimeric configuration (Ahmad et al., 2010; Yousuf et al., 2012).
Catalytic activity of GR and response to abiotic stress
Glutathione reductase undergoes redox interconversion reactions (GSSG to GSH and GSH to GSSG) which depend on the availability of the required substrate (Rao and Reddy, 2008). For every mole of GSSG reduced to GSH, GR requires one mole of NADPH. The enzyme acts like a ping-pong mechanism where a hydride is transferred to FAD as the NADPH binds and it leaves before the di-glutathione binds (Rao and Reddy, 2008). The catalytic mechanism of GR has two phases. The first phase involves the reduction of the flavin moiety by NADPH. GR splits the 2 electrons provided by the reductant NADPH and donates the electrons to each of the two sulfur atoms of GSSG, one at a time. The second phase involves oxidation where the resulting dithiol reacts with GSSG and is reduced to 2 GSH at the active site of the enzyme (Rao and Reddy, 2008; Yousuf et al., 2012). The complete reaction can be represented as:
GR helps to regulate the GSH/GSSG ratio and supplies GSH to guaiacol peroxidase (GPX) and dehydroascorbate reductase (DHAR). GPX helps to remove H2O2 by combining GSH with H2O2 to form H2O and GSSG while DHAR reduces DHA using the GSH to form AA and GSSG (Dumanović et al., 2021).
GR catalyses the last rate limiting step of the Halliwell-Asada (AsA-GSH) pathway and is therefore linked with detoxification of ROS and abiotic stress tolerance in plants (Rao and Reddy, 2008; Hasanuzzaman et al., 2010; Hasanuzzaman et al., 2012). The maintenance of AsA and GSH reduced pools inside the cells is vital for ROS scavenging pathways and performing normal physiological activities. By converting GSSG to GSH, GR helps to maintain this equilibrium and thereby provide stress tolerance in plants (Rao and Reddy, 2008). Additionally, increased GR activity levels help increase the NADP+/NADPH ratio. Thus, the availability of NADP+ to accept electrons from the photosynthetic ETC is ensured, thereby minimizing the formation of ROS species (Mallik et al., 2011). A diagrammatic representation of abiotic stress control by GR is shown in Figure 6. Therefore, an implication of GR in transgenic plants can greatly reduce the ROS induced oxidative stress on the plant and enhance better plant development (Table 2).
Response to drought stress and salinity
Shortages of water and high temperatures that lead to drought-like conditions have serious implications for the cellular machinery of a plant. Drought stress leads to impaired stomatal conductivity, slower rates of electron transport through the membrane transport chain, impaired CO2 diffusion levels, and reduced rates of photosynthesis. All these effects result in significant levels of ROS that cause extensive oxidative damage. Prolonged exposure to drought stress ultimately leads to reduced growth, resulting in lower crop yields (Grover et al., 2011). Several studies have shown an increase in GR activity when plants are exposed to drought stress (Hasanuzzaman et al., 2017). Water scarcity in Ctenanthe setosa results in a characteristic leaf-rolling adaptive response accompanied by increased GSH levels and decreased GSSG levels (Bian and Jiang, 2009; Saruhan et al., 2009). High GSH levels are known to be correlated with water content regulation in leaves (Jiang and Zhang, 2002). Studies have confirmed the effects of elevated GSH levels on drought stress tolerance and the reduction of damages induced by ROS (Bian and Jiang, 2009). GR helps to reduce GSSG to GSH in the presence of NADPH and maintains the reduced GSH pool inside the cell, thereby playing a significant role in stress tolerance. Numerous studies have confirmed the elevated levels of GR activity during drought stress in plants, including barley, maize, wheat, and rice (Kocsy et al., 2002; Chen et al., 2004; Chinnusamy et al., 2005; Selote and Chopra, 2006). A primary effect of drought stress is osmotic stress leading to a sudden change in the solute concentration around the cell and a rapid efflux of water from inside the cell. Kocsy et al. observed that osmotic stress resulted in an increase in GSH levels in wheat seedlings and GR activity in maize (Sumithra et al., 2006). With water scarcity, salinity levels also increase. Saline conditions result in osmotic inhibition and ionic toxicity, affecting normal physiological functions (Demiral and Turkan, 2005; Huang and Guo, 2005b). An increase in GR activity during salinity stress was reported in pea, cantaloupe, soybean, rice, tomato, Arabidopsis thaliana, and wheat (Szalai et al., 2009; Repetto et al., 2012; Hasanuzzaman et al., 2013). These results provide conclusive evidence that GR plays a key role during drought and salt stress in plants.
Response to extreme temperatures
Extremes of temperature, both high and low, are major factors that contribute to poor crop yield and overall plant development. Higher temperatures result in overproduction of ROS, which leads to increased lipid peroxidation, inactivation of the oxygen evolving complex, membrane damage, and DNA damage (Nahar et al., 2015). Similarly, extreme low temperatures also lead to overproduction of ROS due to membrane fluidity degradation, impaired photosynthetic activity, and improper ROS detoxification (Zhang et al., 2008). This highlights the importance of the GSH pools and GSH redox state as vital components in plant thermotolerance. In some maize varieties, GR activity was reported to increase greatly under high temperature (HT) stress treatment (Sumithra et al., 2006). Elevated levels of GSH in wheat at high temperatures suggest the role of GR in thermotolerance (Nahar et al., 2015a). Similar findings were reported in maize and Vigna radiata (Payton et al., 2011). Elevated GSH levels in mustard seedlings suggest efficient eradication of H2O2, thereby confirming increased GR activity (Kuk et al., 2003). High levels of GSH and GR activity were also reported in apple during the reproductive stages, further suggesting their enhanced roles in thermotolerance (Kuk et al., 2003). Low ambient temperature limits the activity of enzymes in the Calvin Cycle, disrupting the sulfhydryl groups and reducing CO2 assimilation (Zitka et al., 2013). Restricted carbon metabolism in the Calvin Cycle leads to insufficient supplies of electron acceptors and overproduction of ROS (Voss et al., 2013). Several studies have shown a positive correlation between cold stress and increased GR activity, including French bean seedlings, rice, and eastern white pine (Peuke and Rennenberg, 2005a; Hasanuzzaman and Fujita, 2013).
Response to heavy metal toxicity
Heavy metals are required for various plant processes and development, but excess heavy metal concentration can lead to toxicity (Peuke and Rennenberg, 2005a). Rapid industrialization has increased heavy metal concentrations in the environment beyond natural sources, disrupting normal physiological growth and generating ROS and oxidative damage (Peuke and Rennenberg, 2005b). To reduce damage and restore normalcy, plants activate various anti-oxidative defense responses, including phytoremediation (Jablonkai, 2022). GSH protects the plant cellular machinery against ROS-oxidative damage in three potential ways: 1) direct quenching of ROS; 2) conjugation of heavy metals and xenobiotic agents to GST; and 3) acting as a precursor for the synthesis of phytochelatins (PCs). By maintaining high levels of PCs, plants can withstand heavy metal stress. GR plays a key role in plant tolerance against heavy metals. GSH is a pivotal factor in the rate-limiting step for phytochelatin formation. The phytochelatins produced form complexes with various heavy metal ions and are sequestered to the vacuole for degradation, thereby limiting oxidative damage (Nahar et al., 2016). Reduced GSH levels are constantly monitored by GR and play a key role in heavy metal stress tolerance. Elevated levels of GR have been reported in Cd-induced stress, and its role in detoxification of ROS via the AsA-GSH cycle has been reported in plants such as radish, soybean, sugarcane, and Arabidopsis thaliana.
Conclusion
Abiotic stresses pose a great challenge for plant growth and development by causing physiological, morphological, and biochemical changes in plant cells. The most common manifestation of abiotic stress is the production of ROS. ROS is both a harmful and beneficial molecule. At low or moderate concentrations, it mediates signal transduction that assists in maintaining cellular homeostasis and facilitates plant acclimatization to stresses. However, its overproduction causes significant damage to plant cells, such as lipid peroxidation, DNA damage, etc. The mechanism for maintaining equilibrium between ROS generation and their quenching involves the production of both enzymatic and non-enzymatic antioxidants. In the last two decades, significant progress has been made in effective ROS scavenging through genetic engineering approaches towards the development of stress-resilient crops. Furthermore, there is a pressing need to identify the genes and understand their mechanisms in the regulation of ROS signaling pathways. Knowledge about the genes and their mechanism of action will definitely help enhance abiotic stress resistance under real agricultural field conditions.
Author contributions
NM and GS provided the fundings. NM and CJ wrote the article. LC and AP gathered the data. AC polished the article.
Funding
This work was supported by grants of the Key Scientific and Technological Grant of Zhejiang for Breeding New Agricultural Varieties (2021C02072-5), Key Research and Development Program of Zhejiang Province (2021C02002) and the International cooperation project of ZAAS (20230012).
Conflict of interest
The authors declare that the research was conducted in the absence of any commercial or financial relationships that could be construed as a potential conflict of interest.
Publisher’s note
All claims expressed in this article are solely those of the authors and do not necessarily represent those of their affiliated organizations, or those of the publisher, the editors and the reviewers. Any product that may be evaluated in this article, or claim that may be made by its manufacturer, is not guaranteed or endorsed by the publisher.
References
Aftab, T., Hakeem, K. R. (2022). Antioxidant defense in plants: molecular basis of regulation (Singapore: Springer).
Agarwal, S. (2007). Increased antioxidant activity in cassia seedlings under UV-b radiation. Biol. Plant 51, 157–160. doi: 10.1007/s10535-007-0030-z
Ahmad, P., Jaleel, C. A., Salem, M. A., Nabi, G., Sharma, S. (2010). Roles of enzymatic and nonenzymatic antioxidants in plants during abiotic stress. Crit. Rev. Biotechnol. 30 (3), 161–175. doi: 10.3109/07388550903524243
Ahmed, I. M., Cao, F., Zhang, M., Chen, X., Zhang, G., Wu, F., et al (2013). Difference in yield and physiological features in response to drought and salinity combined stress during anthesis in Tibetan wild and cultivated barleys. PloS One 8 (10), e77869. doi: 10.1371/journal.pone.0077869
Ajithkumar, I. P., Panneerselvam, R. (2014). ROS scavenging system, osmotic maintenance, pigment and growth status of panicum sumatrense roth. under drought stress. Cell Biochem. Biophys. 68, 587–595. doi: 10.1007/s12013-013-9746-x
Alscher, R. G., Erturk, N., Heath, L. S. (2002). Role of superoxide dismutases (SODs) in controlling oxidative stress in plants. J. Exp. Bot. 53, 1331–1341. doi: 10.1093/jexbot/53.372.1331
Anjum, N. A., Sharma, P., Gill, S. S., Hasanuzzaman, M., Khan, E. A., Kachhap, K., et al. (2016). Catalase and ascorbate peroxidase–representative H2O2-detoxifying heme enzymes in plants. Environ. Sci. pollut. Res. 23, 19002–19029. doi: 10.1007/s11356-016-7309-6
Anjum, S. A., Xie, X., Wang, L. C., Saleem, M. F., Man, C., Lei, W. (2011). Morphological, physiological and biochemical responses of plants to drought stress. Afr. J. Agric. Res. 6 (9), 2026–2032. doi: 10.5897/AJAR10.027
Ansari, M. K. A., Zia, M. H., Ahmad, A., Aref, I. M., Fatma, T., Iqbal, M., et al. (2015). Status of antioxidant defense system for detoxification of arsenic in brassica juncea (L.). Ecoprint: Int. J. Ecol. 22, 7–19. doi: 10.3126/eco.v22i0.15466
Aono, M., Kubo, A., Saji, H., Natori, T., Tanaka, K., Kondo, N. (1993). Enhanced tolerance to photooxidative stress of transgenic nicotiana tabacum with high chloroplastic glutathione reductase activity. Plant Cell Physiol. 34, 129–135. doi: 10.1093/oxfordjournals.pcp.a078386
Apel, K., Hirt, H. (2004). Reactive oxygen species: metabolism, oxidative stress, and signal transduction. Annu. Rev. Plant Biol. 55, 373–399. doi: 10.1146/annurev.arplant.55.031903.141701
Armada, E., Roldán, A., Azcon, R. (2014). Differential activity of autochthonous bacteria in controlling drought stress in native lavandula and salvia plants species under drought conditions in natural arid soil. Microb. Ecol. 67, 410–420. doi: 10.1007/s00248-013-0326-9
Azevedo, R. A., Alas, R. M., Smith, R. J., Lea, P. J. (1998). Response of antioxidant enzymes to transfer from elevated carbon dioxide to air and ozone fumigation, in the leaves and roots of wild-type and a catalase deficient mutant of barley. Physiol. Plant 104, 280–292. doi: 10.1034/j.1399-3054.1998.1040217.x
Bagheri, R., Ahmad, J., Bashir, H., Iqbal, M., Qureshi, M. I.. (2017). Changes in rubisco, cysteine-rich proteins and antioxidant system of spinach (Spinacia oleracea l.) due to sulphur deficiency, cadmium stress and their combination. Protoplasma 254, 1031–1043. doi: 10.1007/s00709-016-1012-9
Bah, A. M., Dai, H., Zhao, J., Sun, H., Cao, F., Zhang, G., et al. (2011). Effects of cadmium, chromium and lead on growth, metal uptake and antioxidative capacity in typha angustifolia. Biol. Trace Elem. Res. 142, 77–92. doi: 10.1007/s12011-010-8746-6
Bauwe, H., Hagemann, M., Kern, R., Timm, S. (2012). Photorespiration has a dual origin and manifold links to central metabolism. Curr. Opin. Plant Biol. 15, 269–275. doi: 10.1016/j.pbi.2012.01.008
Ben-Amor, N., Hamed, K. B., Debez, A., Grignon, C., Abdelly, C. (2005). Physiological and antioxidant response of the perennial halophytes crithmum maritimum to salinity. Plant Sci. 168, 889–899. doi: 10.1016/j.plantsci.2004.11.002
Berwal, M. K., Kumar, R., Prakash, K., Rai, G. K., Hebbar, K. B. (2021). “Antioxidant defense system in plants against abiotic stress,” in Abiotic stress tolerance mechanisms in plants. Eds. Rai, G. K., Kumar, R. R., Bagati, S. (CRC Press), 175–202.
Bian, S., Jiang, Y. (2009). Reactive oxygen species, antioxidant enzyme activities and gene expression patterns in leaves and roots of Kentucky bluegrass in response to drought stress and recovery. Scientia Hortic. 120 (2), 264–270. doi: 10.1016/j.scienta.2008.10.014
Bonifacio, A., Martins, M. O., Ribeiro, C. W., Fontenele, A. V., Carvalho, F. E., Margis-Pinheiro, M., et al. (2011). Role of peroxidases in the compensation of cytosolic ascorbate peroxidase knockdown in rice plants under abiotic stress. Plant Cell Environ. 34, 1705–1722. doi: 10.1111/j.1365-3040.2011.02366.x
Camejo, D., del C. Romero-Puertas, M., Rodríguez-Serrano, M., Sandalio, L. M., Lázaro, J. J., Jiménez, A., et al. (2013). Salinity-induced changes in s-nitrosylation of pea mitochondrial proteins. J. Proteomics 79, 87–99. doi: 10.1016/J.JPROT.2012.12.003
Caverzan, A., Passaia, G., Rosa, S. B., Ribeiro, C. W., Lazzarotto, F., Margis-Pinheiro, M. (2012). Plant responses to stresses: role of ascorbate peroxidase in the antioxidant protection. Genet. Mol. Biol. 35, 1011–1019. doi: 10.1590/S1415-47572012000600016
Chen, K. M., Gong, H. J., Chen, G. C., Wang, S. M., Zhang, C. L. (2004). Gradual drought under field conditions influences the glutathione metabolism, redox balance and energy supply in spring wheat. J. Plant Growth Regul. 23, 20–28. doi: 10.1007/s00344-003-0053-4
Chen, T. H., Murata, N. (2011). Glycinebetaine protects plants against abiotic stress: mechanisms and biotechnological applications. Plant Cell Environ. 34, 1–20. doi: 10.1111/j.1365-3040.2010.02232.x
Chen, Z., Pan, Y. H., An, L. Y., Yang, W. J., Xu, L. G., Zhu, C. (2013). Heterologous expression of a halophilic archaeon manganese superoxide dismutase enhances salt tolerance in transgenic rice. Russian J. Plant Physiol. 60, 359–366. doi: 10.1134/S1021443713030059
Chinnusamy, V., Jagendorf, A., Zhu, J. K. (2005). Understanding and improving salt tolerance in plants. Crop Sci. 45, 437–448. doi: 10.2135/cropsci2005.0437
Corpas, F. J., Fernández-Ocaña, A., Carreras, A., Valderrama, R., Luque, F., Esteban, F. J., et al. (2006). The expression of different superoxide dismutase forms is cell-type dependent in olive (Olea europaea l.) leaves. Plant Cell Physiol. 47, 984–994. doi: 10.1093/pcp/pcj071
Cruz, F. J. R., Castro, G. L. S., Júnior, D. D. S., Pinheiro, H. A. (2013). Exogenous glycine betaine modulates ascorbate peroxidase and catalase activities and prevent lipid peroxidation in mild water stressed carapa guianensis plants. Photosynthetica 51, 102–108. doi: 10.1007/s11099-013-0004-7
Csiszar, J., Lantos, E., Tari, I., Madosa, E., Wodala, B., Vashegyi, A., et al. (2007). Antioxidant enzyme activities in allium species and their cultivars under water stress. Plant Soil Environ. 53, 517–523. doi: 10.17221/2192-PSE
D’Arcy-Lameta, A., Ferrari-Iliou, R., Contour-Ansel, D., Pham-Thi, A. T., Zuily-Fodil, Y. (2006). Isolation and characterization of four ascorbate peroxidase cDNAs responsive to water deficit in cowpea leaves. Ann. Bot. 97, 133–140. doi: 10.1093/aob/mcj010
Das, K., Roychoudhury, A. (2014). Reactive oxygen species (ROS) and response of antioxidants as ROS-scavengers during environmental stress in plants. Front. Environ. Sci. 2. doi: 10.3389/fenvs.2014.00053
Dat, J. F., Pellinen, R., Beeckman, T., Van De Cotte, B., Langebartels, C., Kangasjarvi, J., et al. (2003). Changes in hydrogen peroxide homeostasis trigger an active cell death process in tobacco. Plant J. 33, 621–632. doi: 10.1046/j.1365-313X.2003.01655.x
Deeba, F., Pandey, A. K., Ranjan, S., Mishra, A., Singh, R., Sharma, Y. K., et al. (2012). Physiological and proteomic responses of cotton (Gossypium herbaceum l.) to drought stress. Plant Physiol. Biochem. 53, 6–18. doi: 10.1016/j.plaphy.2012.01.002
Demiral, T., Turkan, I. (2005). Comparative lipid peroxidation, antioxidant defense systems and proline content in roots of two rice cultivars differing in salt tolerance. Environ. Exp. Bot. 53, 247–257. doi: 10.1016/j.envexpbot.2004.03.017
De Pinto, M. C., Locato, V., Sgobba, A., Romero-Puertas, M. D. C., Gadaleta, C., Delledonne, M., et al. (2013). S-nitrosylation of ascorbate peroxidase is part of programmed cell death signaling in tobacco bright yellow-2 cells. Plant Physiol. 163, 1766–1775. doi: 10.1104/pp.113.222703
Diaz-Vivancos, P., Faize, M., Barba-Espin, G., Faize, L., Petri, C., Hernández, J. A., et al. (2013). Ectopic expression of cytosolic superoxide dismutase and ascorbate peroxidase leads to salt stress tolerance in transgenic plums. Plant Biotech. J. 11, 976–985. doi: 10.1111/pbi.12090
Dinakar, C., Djilianov, D., Bartels, D. (2012). Photosynthesis in desiccation tolerant plants: energy metabolism and antioxidative stress defense. Plant Sci. 182, 29–41. doi: 10.1016/j.plantsci.2011.01.018
Doupis, G., Bertaki, M., Psarras, G., Kasapakis, I., Chartzoulakis, K. (2013). Water relations, physiological behavior and antioxidant defence mechanism of olive plants subjected to different irrigation regimes. Sci. Horticult. 153, 150–156. doi: 10.1016/j.scienta.2013.02.010
Dubey, P. K., Singh, G. S., Abhilash, P. C. (2020). Agriculture in a changing climate. adaptive agricultural practices: building resilience in a changing climate (Cham: Springer), 1–10.
Dumanović, J., Nepovimova, E., Natić, M., Kuča, K., Jaćević, V. (2021). The significance of reactive oxygen species and antioxidant defense system in plants: a concise overview. Front. Plant Sci. 11. doi: 10.3389/fpls.2020.552969
Duque, A. S., de Almeida, A. M., da Silva, A. B., da Silva, J. M., Farinha, A. P., Santos, D., et al. (2013). “Abiotic stress responses in plants: unraveling the complexity of genes and networks to survive,” in Abiotic stress: plant responses and applications in agriculture. Eds. Vahdati, K., Lesliepp, C. (In Tech), 49_101.
Ekmekçi, Y., Tanyolac, D., Ayhan, B. (2008). Effects of cadmium on antioxidant enzyme and photosynthetic activities in leaves of two maize cultivars. J. Plant Physiol. 165, 600–611. doi: 10.1016/j.jplph.2007.01.017
Eltayeb, A. E., Kawano, N., Badawi, G. H., Kaminaka, H., Sanekata, T., Shibahara, T., et al. (2007). Overexpression of monodehydroascorbate reductase in transgenic tobacco confers enhanced tolerance to ozone, salt and polyethylene glycol stresses. Planta 225, 1255–1264. doi: 10.1007/s00425-006-0417-7
Eltelib, H. A., Fujikawa, Y., Esaka, M. (2012). Overexpression of the acerola (Malpighia glabra) monodehydroascorbate reductase gene in transgenic tobacco plants results in increased ascorbate levels and enhanced tolerance to salt stress. S. Afr. J. Bot. 78, 295–301. doi: 10.1016/j.sajb.2011.08.005
Faize, M., Burgos, L., Faize, L., Piqueras, A., Nicolas, E., Barba-Espin, G., et al. (2011). Involvement of cytosolic ascorbate peroxidase and Cu/Zn-superoxide dismutase for improved tolerance against drought stress. J. Exp. Bot. 62, 2599–2613. doi: 10.1093/jxb/erq432
Fink, R. C., Scandalios, J. G. (2002). Molecular evolution and structurefunction relationships of the superoxide dismutase gene families in angiosperms and their relationship to other eukaryotic and prokaryotic superoxide dismutases. Arch. Biochem. Biophys. 399, 19–36. doi: 10.1006/abbi.2001.2739
Ford, K. L., Cassin, A., Bacic, A. (2011). Quantitative proteomic analysis of wheat cultivars with differing drought stress tolerance. Front. Plant Sci. 2. doi: 10.3389/fpls.2011.00044
Foyer, C. H., Lelandais, M., Galap, C., Kunert, K. J. (1991). Effects of elevated cytosolic glutathione reductase activity on the cellular glutathione pool and photosynthesis in leaves under normal and stress conditions. Plant Physiol. ogy 97, 863–872. doi: 10.1104/pp.97.3.863
Foyer, C. H., Shigeoka, S. (2011). Understanding oxidative stress and antioxidant functions to enhance photosynthesis. Plant Physiol. 155, 93–100. doi: 10.1104/pp.110.166181
Gao, Y., Guo, Y. K., Lin, S. H., Fang, Y. Y., Bai, J. G. (2010). Hydrogen peroxide pretreatment alters the activity of antioxidant enzymes and protects chloroplast ultrastructure in heat-stressed cucumber leaves. Scientia Hortic. 126 (1), 20–26. doi: 10.1016/j.scienta.2010.06.006
Gepstein, S. H., Glick, B. R. (2013). Strategies to ameliorate abiotic stress-induced plant senescence. Plant Mol. Biol. 82, 623–633. doi: 10.1007/s11103-013-0038-z
Gill, S. S., Anjum, N. A., Hasanuzzaman, M., Gill, R., Trivedi, D. K., Ahmad, I., et al. (2013). Glutathione and glutathione reductase: a boon in disguise for plant abiotic stress defense operations. Plant Physiol. Biochem. 70, 204–212. doi: 10.1016/j.plaphy.2013.05.032
Gill, S. S., Tuteja, N. (2010). Reactive oxygen species and antioxidant machinery in abiotic stress tolerance in crop plants. Plant Physiol. Biochem. 48, 909–930. doi: 10.1016/j.plaphy.2010.08.016
Gomes-Junior, R. A., Moldes, C. A., Delite, F. S., Gratão, P. L., Mazzafera, P. (2006). Nickel elicits a fast antioxidant response in coffea arabica cells. Plant Physiol. Biochem. 44, 420–429. doi: 10.1016/j.plaphy.2006.06.002
Gondim, F. A., Gomes-Filho, E., Costa, J. H., Mendes Alencar, N. L., Prisco, J. T. (2012). Catalase plays a key role in salt stress acclimation induced by hydrogen peroxide pretreatment in maize. Plant Physiol. Biochem. 56, 62–71. doi: 10.1016/j.plaphy.2012.04.012
Grover, A., Singh, A., Blumwald, E. (2011). “Transgenic strategies toward the development of salt-tolerant plants,” in Agricultural salinity assessment and management, 2nd ed. Eds. Wallender, W. W., Tanji, K. K. (Reston, VA, USA: American Society of Civil Engineers), 235–274.19.
Guan, Q., Wang, Z., Wang, X., Takano, T., Liu, S. (2015). A peroxisomal APX from puccinellia tenuiflora improves the abiotic stress tolerance of transgenic arabidopsis thaliana through decreasing of H2O2 accumulation. J. Plant Physiol. 175, 183–191. doi: 10.1016/j.jplph.2014.10.020
Hajam, Y. A., Lone, R., Kumar, R. (2023). “Role of plant phenolics against reactive oxygen species (ROS) induced oxidative stress and biochemical alterations,” in Plant phenolics in abiotic stress management. Eds. Lone, R., Khan, S., Mohammed Al-Sadi, A. (Singapore: Springer). doi: 10.1007/978-981-19-6426-8_7
Hasanuzzaman, M., Fujita, M. (2013). Exogenous sodium nitroprusside alleviates arsenic-induced oxidative stress in wheat (Triticum aestivum l.) seedlings by enhancing antioxidant defense and glyoxalase system. Ecotoxicology 22, 584–596. doi: 10.1007/s10646-013-1050-4
Hasanuzzaman, M., Hossain, M. A., Fujita, M. (2010). Physiological and biochemical mechanisms of nitric oxide induced abiotic stress tolerance in plants. Am. J. Plant Physiol. 5. doi: 10.3923/ajpp.2010.295.324
Hasanuzzaman, M., Hossain, M., Silva, J. T., Fujita, M. (2012). “Plant response and tolerance to abiotic oxidative stress: antioxidant defense is a key factor,” in Crop stress and its management: perspectives and strategies. Eds. Venkateswarlu, B., Shanker, A. K., Shanker, C., Maheswari, M. (Netherlands: Springer), 261e315.
Hasanuzzaman, M., Nahar, K., Alam, M., Roychowdhury, R., Fujita, M. (2013). Physiological, biochemical, and molecular mechanisms of heat stress tolerance in plants. Int. J. Mol. Sci. 14, 9643–9684. doi: 10.3390/ijms14059643
Hasanuzzaman, M., Nahar, K., Anee, T. I., Fujita, M. (2017). Glutathione in plants: biosynthesis and physiological role in environmental stress tolerance. Physiol. Mol. Biol. Plants 23 (2), 249–268. doi: 10.1007/s12298-017-0422-2
Hernandez, J. A., del Rıo, L. A., Sevilla, F. (2006). Salt stress-induced changes in superoxide dismutase isozymes in leaves and mesophyll protoplasts from vigna unguiculata (L.) walp. New Phytology 126, 37–44. doi: 10.1111/j.1469-8137.1994.tb07527.x
Hong, C. Y., Hsu, Y. T., Tsai, Y. C., Kao, C. H. (2007). Expression of ascorbate peroxidase 8 in roots of rice (Oryza sativa l.) seedlings in response to NaCl. J. Exp. Bot. 58, 3273–3283. doi: 10.1093/jxb/erm174
Huang, M., Guo, Z. (2005a). Responses of antioxidative system to chilling in two rice cultivars differing in sensitivity. Biol. Plant 49, 81–84. doi: 10.1007/s00000-005-1084-3
Huang, M., Guo, Z. (2005b). Responses of antioxidative system to chilling stress in two rice cultivars differing in sensitivity. Biol. Plant 49, 81–84. doi: 10.1007/s00000-005-1084-3
Husen, A., Iqbal, M., Sohrab, S. S., Ansari, M. K. A. (2018). Salicylic acid alleviates salinity-caused damage to foliar functions, plant growth and antioxidant system in Ethiopian mustard (Brassica carinata a. br.). Agric. Food Secur 7, 44. doi: 10.1186/s40066-018-0194-0
Hussain, A., Tanveer, M., Brestic, M., Fatma, M., Iqbal, N., Hashem, A. (2020). Reactive oxygen species and abiotic stress tolerance in plants. Plant Cell Rep. 39 (3), 281–292.
Ishikawa, T., Morimoto, Y., Madhusudhan, R., Sawa, Y., Shibata, H., Yabuta, Y., et al. (2005). Acclimation to diverse environmental stresses caused by a suppression of cytosolic ascorbate peroxidase in tobacco BY-2 cells. Plant Cell Physiol. 46, 1264–1271. doi: 10.1093/pcp/pci135
Jablonkai, I. (2022). “Molecular defense mechanisms in plants to tolerate toxic action of heavy metal environmental pollution,” in Plant defense mechanisms, June (IntechOpen). doi: 10.5772/intechopen.102330
Jaleel, C. A., Manivannan, P., Kishorekumar, A., Sankar, B., et al. (2007). Alterations in osmoregulation, antioxidant enzymes and indole alkaloid levels in catharanthus roseus exposed to water deficit. Colloids Surf B: Biointerfaces 59, 150–157. doi: 10.1016/j.colsurfb.2007.05.001
Jia, X., Wang, W. X., Ren, L., Chen, Q. J., Mendu, V., Willcut, B., et al. (2009). Differential and dynamic regulation of miR398 in response to ABA and salt stress in populus tremula and arabidopsis thaliana. Plant Mol. Biol. 71, 51–59. doi: 10.1007/s11103-009-9508-8
Jiang, M., Zhang, J. (2002). Water stress-induced abscisic acid accumulation triggers the increased generation of reactive oxygen species and up-regulates the activities of antioxidant enzymes in maize leaves. J. Exp. Bot. 53, 2401–2410. doi: 10.1093/jxb/erf090
Khan, I., Ahmad, A., Iqbal, M. (2009). Modulation of antioxidant defense system for arsenic detoxification in Indian mustard. Ecotoxic. Environ. Saf. 72, 626–634. doi: 10.1016/j.ecoenv.2007.11.016
Khan, M. I., Fatma, M., Per, T. S., Anjum, N. A., Khan, N. A. (2015). Salicylic acid-induced abiotic stress tolerance and underlying mechanisms in plants. Front. Plant Sci. 6, 462. doi: 10.3389/fpls.2015.00462
Khan, A., Numan, M., Khan, A. L., Lee, I.-J., Imran, M., Asaf, S., et al. (2020). Melatonin: awakening the defense mechanisms during plant oxidative stress. Plants 9 (4), 407. doi: 10.3390/plants9040407
Khatun, S., Babar, M., Hahn, E., Paek, K. (2008). Copper toxicity in withania somnifera : growth and antioxidant enzymes responses of in vitro grown plants. J. Environ. Exp. Bot. 64, 279–285. doi: 10.1016/j.envexpbot.2008.02.004
Kocsy, G., Szalai, G., Galiba, G. (2002). Induction of glutathione synthesis and glutathione reductase activity by abiotic stresses in maize and wheat. Sci. World J. 2, 1726–1732. doi: 10.1100/tsw.2002.812
Kornyeyev, D., Logan, B. A., Payton, P., Allen, R. D., Holaday, A. S. (2001). Enhanced photochemical light utilization and decreased chilling induced photoinhibition of photosystem II in cotton over expressing genes encoding chloroplast targeted antioxidant enzymes. Physiol. Plant 113, 323–331. doi: 10.1034/j.1399-3054.2001.1130304.x
Koussevitzky, S., Suzuki, N., Huntington, S., Armijo, L., Sha, W., Cortes, D., et al. (2008). Ascorbate peroxidase 1 plays a key role in the response of arabidopsis thaliana to stress combination. J. Biol. Chem. 283, 34197–34203. doi: 10.1074/jbc.M806337200
Kuk, Y. I., Shin, J. S., Burgos, N. R., Hwang, T. E., Han, O., Cho, B. H., et al. (2003). Antioxidative enzymes offer protection from chilling damage in rice plants. Crop Sci. 43, 2109–2117. doi: 10.2135/cropsci2003.2109
Kumar, N., Singh, H., Sharma, S. K. (2020). “Antioxidants: responses and importance in plant defense system,” in Sustainable agriculture in the era of climate change. Eds. Roychowdhury, R., Choudhury, S., Hasanuzzaman, M., Srivastava, S. (Cham: Springer), 251–264.
Kumar, D., Yusuf, M. A., Singh, P., Sardar, M., Sarin, N. B. (2013). Modulation of antioxidant machinery in α-tocopherol-enriched transgenic brassica juncea plants tolerant to abiotic stress conditions. Protoplasma 250, 1079–1089. doi: 10.1007/s00709-013-0484-0
Kwon, S., Jeong, Y., Lee, H., Kim, J., Cho, K., Allen, R., et al. (2002). Enhanced tolerances of transgenic tobacco plants expressing both superoxide dismutase and ascorbate peroxidase in chloroplasts against methyl viologen mediated oxidative stress. Plant Cell Environ. 25, 873–882. doi: 10.1046/j.1365-3040.2002.00870.x
Latef, A. A. H. A. (Ed.) (2021). Organic solutes, oxidative stress, and antioxidant enzymes under abiotic stressors. 1st ed (CRC Press). doi: 10.1201/9781003022879
Lazzarotto, F., Teixeira, F. K., Rosa, S. B., Dunand, C., Fernandes, C. L., de Vasconcelos Fontenele, A., et al. (2011). Ascorbate peroxidaserelated (APx-r) is a new heme-containing protein functionally associated with ascorbate peroxidase but evolutionarily divergent. New Phytol. 191, 234–250. doi: 10.1111/j.1469-8137.2011.03659.x
Lee, Y. P., Ahmad, R., Lee, H. S., Kwak, S. S., Shafqat, M. N., Kwon, S. Y. (2013). Improved tolerance of Cu/Zn superoxide dismutase and ascorbate peroxidase expressing transgenic tobacco seeds and seedlings against multiple abiotic stresses. Int. J. Agric. Biol. 15, 725–730.
Lee, S. H., Ahsan, N., Lee, K. W., Kim, D. H., Lee, D. G., Kwak, S. S., et al. (2007). Simultaneous overexpression of both CuZn superoxide dismutase and ascorbate peroxidase in transgenic tall fescue plants confers increased tolerance to a wide range of abiotic stresses. J. Plant Physiol. 164, 1626–1638. doi: 10.1016/j.jplph.2007.01.003
Li, Y. J., Hai, R. L., Du, X. H., Jiang, X. N., Lu, H. (2009). Overexpression of a populus peroxisomal ascorbate peroxidase (PpAPX) gene in tobacco plants enhances stress tolerance. Plant Breed. 128, 404–410. doi: 10.1111/j.1439-0523.2008.01593.x
Lim, S., Kim, Y. H., Kim, S. H., Kwon, S. Y., Lee, H. S., Kim, J. S., et al. (2007). Enhanced tolerance of transgenic sweetpotato plants that express both CuZnSOD and APX in chloroplasts to methyl viologen-mediated oxidative stress and chilling. Mol. Breed. 19, 227–239. doi: 10.1007/s11032-006-9051-0
Lu, Z., Liu, D., Liu, S. (2007). Two rice cytosolic ascorbate peroxidases differentially improve salt tolerance in transgenic arabidopsis. Plant Cell Rep. 26, 1909–1917. doi: 10.1007/s00299-007-0395-7
Mahanty, S., Kaul, T., Pandey, P., Reddy, R. A., Mallikarjuna, G., Reddy, C. S., et al. (2012). Biochemical and molecular analyses of copper_zinc superoxide dismutase from a C4 pennisetum glaucum reveals an adaptive role in response to oxidative stress. Gene 505, 309–317. doi: 10.1016/j.gene.2012.06.001
Malar, S., Vikram, S. S., Favas, P. J. C., Perumal, V. (2014). Lead heavy metal toxicity induced changes on growth and antioxidative enzymes level in water hyacinths [Eichhornia crassipes (Mart.)]. Bot. Stud. 55, 54. doi: 10.1186/s40529-014-0054-
Mallik, S., Nayak, M., Sahu, B. B., Panigrahi, A. K., Shaw, B. P. (2011). Response of antioxidant enzymes to high NaCl concentration in different salt-tolerant plants. Biol. Plant 55, 191–195. doi: 10.1007/s10535-011-0029-3
Markovska, Y. K., Gorinova, N. I., Nedkovska, M. P., Miteva, K. M. (2009). Cadmium-induced oxidative damage and antioxidant responses in brassica juncea plants. Biol. Plant 53, 151–154. doi: 10.1007/s10535-009-0023-1
Maruta, T., Tanouchi, A., Tamoi, M., Yabuta, Y., Yoshimura, K., Ishikawa, T., et al. (2010). Arabidopsis chloroplastic ascorbate peroxidase isoenzymes play a dual role in photoprotection and gene regulation under photooxidative stress. Plant Cell Physiol. 51 (2), 190–200. doi: 10.1093/pcp/pcp177
Matos, I. F., Morales, L. M. M., Santana, D. B., Silva, G. M. C., Gomes, M. M. A., Ayub, R. A., et al. (2022). Ascorbate synthesis as an alternative electron source for mitochondrial respiration: possible implications for the plant performance. Front. Plant Sci. 13. doi: 10.3389/fpls.2022.987077
Mhamdi, A., Queval, G., Chaouch, S., Vanderauwera, S., Van Breusegem, F., Noctor, G. (2010). Catalase function in plants: a focus on arabidopsis mutants as stress-mimic models. J. Exp. Bot. 61 (15), 4197–4220. doi: 10.1093/jxb/erq282
Miller, G., Suzuki, N., Rizhsky, L., Hegie, A., Koussevitzky, S., Mittler, R. (2007). Double mutants deficient in cytosolic and thylakoid ascorbate peroxidase reveal a complex mode of interaction between reactive oxygen species, plant development, and response to abiotic stresses. Plant Physiol. 144, 1777–1785. doi: 10.1104/pp.107.101436
Minhas, P. S., Rane, J., Pasala, R. K. (2017). “Abiotic stresses in agriculture: an overview,” in Abiotic stress management for resilient agriculture (Singapore: Springer), 3–8.
Mishra, P., Bhoomika, K., Dubey, R. S. (2013). Differential responses of antioxidative defense system to prolonged salinity stress in salttolerant and salt-sensitive indica rice (Oryza sativa l.) seedlings. Protoplasma 250, 3–19. doi: 10.1007/s00709-011-0365-3
Mittler, R. (2002). Oxidative stress, antioxidants and stress tolerance. Trends Plant Sci. 7, 405–410. doi: 10.1016/S1360-1385(02)02312-9
Mittler, R. (2006). Abiotic stress, the field environment and stress combination. Trends Plant Sci. 11, 15–19. doi: 10.1016/j.tplants.2005.11.002
Mittler, R., Vanderauwera, S., Suzuki, N., Miller, G., Tognetti, V. B., Vandepoele, K., et al. (2011). ROS signaling: the new wave? Trends Plant Sci. 16, 300–309. doi: 10.1016/j.tplants.2011.03.007
Mutlu, S., Karadagoglu, O., Atici, O., Nalbantoglu, B. (2013). Protective role of salicylic acid applied before cold stress on antioxidative system and protein patterns in barley apoplast. Biol. Plantarum 57, 507–513. doi: 10.1007/s10535-013-0322-4
Mylona, P. V., Polidoros, A. N. (2010). “ROS regulation of antioxidant genes,” in Reactive oxygen species and antioxidants in higher plants. Ed. Gupta, S. D. (Science Publishers), 101–127.
Mylona, P. V., Polidoros, A. N., Scandalios, J. G. (2007). Antioxidant gene responses to ROS-generating xenobiotics in developing and germinated scutella of maize. J. Exp. Bot. 58, 1301–1312. doi: 10.1093/jxb/erl292
Nagajyoti, P. C., Lee, K. D., Sreekanth, T. V. M. (2010). Heavy metals, occurrence and toxicity for plants: a review. Environ. Chem. Lett. 8, 199–216. doi: 10.1007/s10311-010-0297-8
Nageshbabu, R., Jyothi, M. N. (2013). Profile of small interfering RNAs from french bean phaseolus vulgaris under abiotic stress conditions. Int. J. Pharm. Bio. Sci. 4, 176–185.
Nahar, K., Hasanuzzaman, M., Alam, M. M., Fujita, M. (2015). Glutathione-induced drought stress tolerance in mung bean: coordinated roles of the antioxidant defence and methylglyoxal detoxification systems. AoB Plants. doi: 10.1093/aobpla/plv069
Nahar, K., Rahman, M., Hasanuzzaman, M., Alam, M. M., Rahman, A., Suzuki, T., et al. (2016). Physiological and biochemical mechanisms of spermine-induced cadmium stress tolerance in mung bean (Vigna radiata l.) seedlings. Environ. Sci. pollut. Res. Int. 23 (21), 21206–21218. doi: 10.1007/s11356-016-7295-8
Najami, N., Janda, T., Barriah, W., Kayam, G., Tal, M., Guy, M., et al. (2008). Ascorbate peroxidase gene family in tomato: its identification and characterization. Mol. Genet. Genomics 279, 171–182. doi: 10.1007/s00438-007-0305-2
Nakano, T., Suzuki, K., Fujimura, T. (2006). Shinkei-kasokuka (SK) genes encode previously unknown zinc finger proteins involved in oxidative stress response in yeast. J. Biol. Chem. 281 (39), 28389–28397.
Narendra, S., Venkataramani, S., Shen, G., Wang, J., Pasapula, V., Lin, Y., et al. (2006). The arabidopsis ascorbate peroxidase 3 is a peroxisomal membranebound antioxidant enzyme and is dispensable for arabidopsis growth and development. J. Exp. Bot. 57, 3033–3042. doi: 10.1093/jxb/erl060
Nareshkumar, A., Veeranagamallaiah, G., Pandurangaiah, M., Kiranmai, K., Amaranathareddy, V., Lokesh, U., et al. (2015). Pb-Stress induced oxidative stress caused alterations in antioxidant efficacy in two groundnut (Arachis hypogaea l.) cultivars. Agric. Sci. 6, 1283–1297. doi: 10.4236/as.2015.610123
Nawaz, M., Anjum, S. A., Ashraf, U., Azeem, F., Wang, Z. (2021). “Antioxidant defense system and reactive oxygen species (ROS) interplay in plants under drought condition,” in Handbook of climate change management. Eds. Luetz, J. M., Ayal, D. (SpringerCham), 93–117. doi: 10.1007/978-3-030-57281-5_121
Nawaz, M., Wang, Z. (2020). Abscisic acid and glycine betaine mediated tolerance mechanisms under drought stress and recovery in axonopus compressus: a new insight. Sci. Rep. 10, 6942. doi: 10.1038/s41598-020-63447-0
Nazar, R., Iqbal, N., Syeed, S., Khan, N. A. (2011). Salicylic acid alleviates decreases in photosynthesis under salt stress by enhancing nitrogen and sulfur assimilation and antioxidant metabolism differentially in two mungbean cultivars. J. Plant Physiol. 168, 807–815. doi: 10.1016/j.jplph.2010.11.001
Nouairi, I., Ammar, W. B., Youssef, N. B., Miled, D. D. B., Ghorbal, M. H., Zarrouk, M. (2009). Antioxidant defense system in leaves of Indian mustard (Brassica juncea) and rape (Brassica napus) under cadmium stress. Acta Physiol. Plant 31, 237–247. doi: 10.1007/s11738-008-0224-9
Nounjan, N., Nghia, P. T., Theerakulpisut, P. (2012). Exogenous proline and trehalose promote recovery of rice seedlings from salt-stress and differentially modulate antioxidant enzymes and expression of related genes. J. Plant Physiol. 169, 596–604. doi: 10.1016/j.jplph.2012.01.004
Oliva, M., Theiler, G., Zamocky, M., Koua, D., Margis-Pinheiro, M., Passardi, F., et al. (2009). PeroxiBase: a powerful tool to collect and analyse peroxidase sequences from viridiplantae. J. Exp. Bot. 60, 453–459. doi: 10.1093/jxb/ern317
Pan, Y., Wu, L. J., Yu, Z. L. (2006). Effect of salt and drought stress on antioxidant enzymes activities and SOD isoenzymes of liquorice (Glycyrrhiza uralensis fisch). Plant Growth Regul. 49, 157–165. doi: 10.1007/s10725-006-9101-y
Panda, S. K., Matsumoto, H. (2010). Changes in antioxidant gene expression and induction of oxidative stress in pea (Pisum sativum l.) under Al stress. Biometals 23, 753–762. doi: 10.1007/s10534-010-9342-0
Park, S. Y., Ryu, S. H., Jang, I. C., Kwon, S. Y., Kim, J. G., Kwak, S. S. (2004). Molecular cloning of a cytosolic ascorbate peroxidase cDNA from cell cultures of sweetpotato and its expression in response to stress. Mol. Genet. Genomics 271, 339–346. doi: 10.1007/s00438-004-0986-8
Passardi, F., Bakalovic, N., Teixeira, F. K., Margis-Pinheiro, M., Penel, C., Dunand, C. (2007). Prokaryotic origins of the nonanimal peroxidase superfamily and organelle-mediated transmission to eukaryotes. Genomics 89, 567–579. doi: 10.1016/j.ygeno.2007.01.006
Payton, P., Webb, R., Kornyeyev, D., Allen, R., Holaday, A. S. (2011). Protecting cotton photosynthesis during moderate chilling at high light intensity by increasing chloroplastic antioxidant enzyme activity. J. Exp. Bot. 52, 2345–2354. doi: 10.1093/jexbot/52.365.2345
Peng, H. Y., Yang, X. E., Yang, M. J., Tian, S. K. (2006). Responses of antioxidant enzyme system to copper toxicity and copper detoxification in the leaves of elsholtzia splendens. J. Plant Nutr. 29, 1619–1635. doi: 10.1080/01904160600851478
Peñuelas, J., Munné-Bosch, S. (2005). Isoprenoids: an evolutionary pool for photoprotection. Trends Plant Sci. 10, 166–169. doi: 10.1016/j.tplants.2005.02.005
Peuke, A. D., Rennenberg, H. (2005a). Phytoremediation with transgenic trees. Z Naturforsch. 60, 199–207.
Peuke, A. D., Rennenberg, H. (2005b). Phytoremediation: molecular biology, requirements for application, environmental protection, public attention and feasibility. EMBO Rep. 6, 497–501. doi: 10.1038/sj.embor.7400445
Pinheiro, C., Chaves, M. M. (2011). Photosynthesis and drought: can we make metabolic connections from available data? J. Exp. Bot. 62, 869–882. doi: 10.1093/jxb/erq340
Pinto, A. P., Alves, A. S., Candeias, A. J., Cardoso, A. I., de Varennes, A., Martins, L. L., et al. (2009). Cadmium accumulation and antioxidative defenses in brassica juncea l. czern, nicotiana tabacum l. and solanum nigrum l. Int. J. Environ. Anal. Chem. 89, 661–676. doi: 10.1080/03067310902962585
Pourcel, L., Routaboul, J. M., Cheynier, V., Lepiniec, L., Debeaujon, I. (2007). Flavonoid oxidation in plants: from biochemical properties to physiological functions. Trends Plant Sci. 12, 29–36. doi: 10.1016/j.tplants.2006.11.006
Rahman, S., Iqbal, M., Husen, A. (2023). “Medicinal plants and abiotic stress: an overview,” in Medicinal plants. Eds. Husen, A., Iqbal, M. (Singapore: Springer). doi: 10.1007/978-981-19-5611-9_1
Rajput, V. D., Singh, R. K., Verma, K. K., Sharma, L., Quiroz-Figueroa, F. R., Meena, M., et al. (2021). Recent developments in enzymatic antioxidant defence mechanism in plants with special reference to abiotic stress. Biology 10 (4), 267. doi: 10.3390/biology10040267
Ramakrishna, A., Gill, S. S. (2018). Metabolic adaptations in plants during abiotic stress. CRC Press Boca Raton, 442.
Rao, A. S. V. C., Reddy, A. R. (2008). “Glutathione reductase: a putative redox regulatory system in plant cells,” in Sulfur assimilation and abiotic stress in plants. Eds. Khan, N. A., Singh, S., Umar, S. (Berlin: Springer). doi: 10.1007/978-3-540-76326-0_6
Rasool, S., Ahmad, A., Siddiqi, T. O., Ahmad, P. (2013). Changes in growth, lipid peroxidation and some key antioxidant enzymes in chickpea genotypes under salt stress. Acta Physiologia Plantarum 35, 1039–1050. doi: 10.1007/s11738-012-1142-4
Repetto, M., Semprine, J., Boveris, A. (2012). “Lipid peroxidation: chemical mechanism, biological implications and analytical determination,” in Lipid peroxidation. Ed. Catala, A. (Rijeka, Croatia: InTech), 3–30.
Rosa, S. B., Caverzan, A., Teixeira, F. K., Lazzarotto, F., Silveira, J. A., Ferreira-Silva, S. L., et al. (2010). Cytosolic APX knockdown indicates an ambiguous redox response in rice. Phytochemistry 71, 548–558. doi: 10.1016/j.phytochem.2010.01.003
Rossa, M. M., de Oliveira, M. C., Okamoto, O. K., Lopes, P. F., Colepicolo, P. (2002). Effect of visible light on superoxide dismutase (SOD) activity in the red alga Gracilariopsis tenuifrons (Gracilariales, rhodophyta). J. Appl. Phycology 14, 151–157. doi: 10.1023/A:1019985722808
Roychoudhury, A., Ghosh, S. (2013). Physiological and biochemical responses of mungbean (Vigna radiata l. wilczek) to varying concentrations of cadmium chloride or sodium chloride. Unique J. Pharm. Biol. Sci. 1, 11–21.
Sairam, R. K., Shukla, D. S., Saxena, D. C. (1998). Stress-induced injury and antioxidant enzymes in relation to drought tolerance in wheat genotypes. Biol. Plant 40, 357–364. doi: 10.1023/A:1001009812864
Sairam, R. K., Srivastava, G. C., Agarwal, S., Meena, R. C. (2005). Differences in antioxidant activity in response to salinity stress in tolerant and susceptible wheat genotypes. Biol. Plantarum 49, 85–91. doi: 10.1007/s10535-005-5091-2
Saruhan, N., Terzi, R., Saglam, A., Kadioglu, A. (2009). The relationship between leaf rolling and ascorbate-glutathione cycle enzymes in apoplastic and symplastic areas of ctenanthe setosa subjected to drought stress. Biol. Res. 42, 315–326. doi: 10.4067/S0716-97602009000300006
Sato, Y., Masuta, Y., Saito, K. (2011). Enhanced chilling tolerance at the booting stage in rice by transgenic overexpression of the ascorbate peroxidase gene, OsAPXa. Plant Cell Rep. 30, 399–406. doi: 10.1007/s00299-010-0985-7
Secenji, M., Hideg, É., Bebes, A., Györgyey, J. (2009). Trans ˇ criptional differences in gene families of the ascorbate-glutathione cycle in wheat during mild water deficit. Plant Cell Rep. 29, 37–50. doi: 10.1007/s00299-009-0796-x
Sekmen, A. H., Türkan, I., Takio, S. (2007). Differential responses of antioxidative enzymes and lipid peroxidation to salt stress in salt-tolerant plantago maritima and salt-sensitive plantago media. Physiol. Plant 131, 399–411. doi: 10.1111/j.1399-3054.2007.00970.x
Selote, D. S., Chopra, R. K. (2006). Drought acclimation confers oxidative stress tolerance by inducing co-ordinated antioxidant defense at cellular and subcellular level in leaves of wheat seedlings. Physiol. Plant 127, 494–450. doi: 10.1111/j.1399-3054.2006.00678.x
Sen, A., Alikamanoglu, S. (2013). Antioxidant enzyme activities, malondialdehyde, and total phenolic content of PEG-induced hyperhydric leaves in sugar beet tissue culture. In Vitro Cell. Dev. Biology-Plant 49, 396–404. doi: 10.1007/s11627-013-9511-2
Shao, H. B., Chu, L. Y., Wu, G., Zhang, J. H., Lu, Z. H., Hu, Y. C. (2007). Changes of some anti-oxidative physiological indices under soil water deficits among 10 wheat (Triticum aestivum) genotypes at tillering stage. Biointerfaces 59, 113–119. doi: 10.1016/j.colsurfb.2006.09.004
Sharma, P., Dubey, R. S. (2005). Drought induces oxidative stress and enhances the activities of antioxidant enzymes in growing rice seedlings. Plant Growth Regul. 46, 209–221. doi: 10.1007/s10725-005-0002-2
Sharma, P., Dubey, R. S. (2007). Involvement of oxidative stress and role of antioxidative defense system in growing rice seedlings exposed to toxic concentrations of aluminum. Plant Cell Rep. 26, 2027–2038. doi: 10.1007/s00299-007-0416-6
Sharma, P., Jha, A. B., Dubey, R. S., Pessarakli, M. (2012). Reactive oxygen species, oxidative damage, and antioxidative defense mechanism in plants under stressful conditions. J. Bot., 1–26. doi: 10.1155/2012/217037
Sheokand, S., Kumari, A., Sawhney, V. (2008). Effect of nitric oxide and putrescine on antioxidative responses under NaCl stress in chickpea plants. Physiol. Mol. Biol. Plants 14, 355–362. doi: 10.1007/s12298-008-0034-y
Siddiqui, Z. S. (2013). Effects of double stress on antioxidant enzyme activity in vigna radiata (L.) wilczek. Acta Bot. Croatica 72, 145–156. doi: 10.2478/v10184-012-0011-y
Singh, N., Mishra, A., Jha, B. (2014). Ectopic over-expression of peroxisomal ascorbate peroxidase (SbpAPX) gene confers salt stress tolerance in transgenic peanut (Arachis hypogaea). Gene 547, 119–125. doi: 10.1016/j.gene.2014.06.037
Smeets, K., Ruytinx, J., Semane, B., Van Belleghem, F., Remans, T., Van Sanden, S., et al. (2008). Cadmium-induced transcriptional and enzymatic alterations related to oxidative stress. Environ. Exp. Bot. 63, 1–8. doi: 10.1016/j.envexpbot.2007.10.028
Sobhanian, H., Aghaei, K., Komatsu, S. (2011). Changes in the plant proteome resulting from salt stress: toward the creation of salt-tolerant crops? J. Proteomics 74, 1323–1337. doi: 10.1016/j.jprot.2011.03.018
Sofo, A., Dichio, B., Xiloyannis, C., Masia, A. (2005a). Antioxidant defences in olive tree during drought stress: changes in activity of some antioxidant enzymes. Funct. Plant Biol. 32, 45–53. doi: 10.1071/FP04003
Sofo, A., Tuzio, A. C., Dichio, B., Xiloyannis, C. (2005b). Influence of water deficit and rewatering on the components of the ascorbate – glutathione cycle in four interspecific prunus hybrids. Plant Sci. 169, 403–412. doi: 10.1016/j.plantsci.2005.04.004
Song, X. S., Hu, W. H., Mao, W. H., Ogweno, J. O., Zhou, Y. H., Yu, J. Q. (2005). Response of ascorbate peroxidase isoenzymes and ascorbate regeneration system to abiotic stresses in cucumis sativus l. Plant Physiol. Biochem. 43, 1082–1088. doi: 10.1016/j.plaphy.2005.11.003
Sorkheh, K., Shiran, B., Khodambashi, M., Rouhi, V., Mosavei, S., Sofo, A. (2012a). Exogenous proline alleviates the effects of H2O2-induced oxidative stress in wild almond species (Prunus spp.). Russ. J. Plant Physiol. 59, 788–798. doi: 10.1134/S1021443712060167
Sorkheh, K., Shiran, B., Rouhi, V., Khodambashi, M., Sofo, A. (2012b). Salt stress induction of some key antioxidant enzymes and metabolites in eight Iranian wild almond species. Acta Physiol. Plant 34, 203–213. doi: 10.1007/s11738-011-0819-4
Srivalli, B., Chinnusamy, V., Khanna-Chopra, R. (2003). Antioxidant defense in response to abiotic stresses in plants. J. Plant Biol. 30, 121–139.
Sumithra, K., Jutur, P. P., Dalton, C. B., Reddy, A. R. (2006). Salinity-induced changes in two cultivars of vigna radiata: responses of antioxidative and proline metabolism. Plant Growth Regul. 50, 11–22. doi: 10.1007/s10725-006-9121-7
Sun, W. H., Duan, M., Shu, D. F., Yang, S., Meng, Q. W. (2010). Overexpression of StAPX in tobacco improves seed germination and increases early seedling tolerance to salinity and osmotic stresses. Plant Cell Rep. 29, 917–926. doi: 10.1007/s00299-010-0878-9
Sunkar, R., Kapoor, A., Zhu, J. K. (2006). Post-transcriptional induction of two Cu/Zn superoxide dismutase genes in arabidopsis is mediated by down-regulation of miR398 and important for oxidative stress tolerance. Plant Cell 18, 2051–2065. doi: 10.1105/tpc.106.041673
Szalai, G., Kellos, T., Galiba, G., Kocsy, G. (2009). Glutathione as an antioxidant and regulatory molecule in plantsunder abiotic stress conditions. Plant Growth Regul. 28, 66–80. doi: 10.1007/s00344-008-9075-2
Szepesi, A., Poor, P., Gémes, K., Horvath, E., Tari, I. (2008). Influence of exogenous salicylic acid on antioxidant enzyme activities in the roots of salt stressed tomato plants. Acta Biologica Szegediensis 52, 199–200.
Tang, L., Kwon, S. Y., Kim, S. H., Kim, J. S., Choi, J. S., Cho, K. Y., et al. (2006). Enhanced tolerance of transgenic potato plants expressing both superoxide dismutase and ascorbate peroxidase in chloroplasts against oxidative stress and high temperature. Plant Cell Rep. 25, 1380–1386. doi: 10.1007/s00299-006-0199-1
Tanou, G., Filippou, P., Belghazi, M., Job, D., Diamantidis, G., Fotopoulos, V., et al. (2012). Oxidative and nitrosative-based signaling and associated post-translational modifications orchestrate the acclimation of citrus plants to salinity stress. Plant J. 72, 585–599. doi: 10.1111/j.1365-313X.2012.05100.x
Tari, I., Camen, D., Coradini, G., Csiszar, J., Fediuc, E., Gémes, K., et al. (2008). Changes in chlorophyll fluorescence parameters and oxidative stress responses of bush bean genotypes for selecting contrasting acclimation strategies under water stress. Acta Biologica Hungarica 59, 335–345. doi: 10.1556/ABiol.59.2008.3.7
Teixeira, F. K., Menezes-Benavente, L., Galvão, V. C., Margis, R., Margis-Pinheiro, M. (2006). Rice ascorbate peroxidase gene family encodes functionally diverse isoforms localized in different subcellular compartments. Planta 224, 300–314. doi: 10.1007/s00425-005-0214-8
Teixeira, F. K., Menezes-Benavente, L., Galvão, V. C., MargisPinheiro, M. (2005). Multigene families encode enzymes of antioxidant metabolism in eucalyptus grandis l. Genet. Mol. Biol. 28, 529–538. doi: 10.1590/S1415-47572005000400007
Teixeira, F. K., Menezes-Benavente, L., Margis, R., Margis Pinheiro, M. (2004). Analysis of the molecular evolutionary history of the ascorbate peroxidase gene family: inferences from the rice genome. J. Mol. Evol. 59, 761–770. doi: 10.1007/s00239-004-2666-z
Tertivanidis, K., Goudoula, C., Vasilikiotis, C., Hassiotou, E., Perl-Treves, R., Tsaftaris, A. (2004). Superoxide dismutase transgenes in sugar beets confer resistance to oxidative agents and the fungus c. beticola. Transgenic Res. 13, 225–233. doi: 10.1023/B:TRAG.0000034610.35724.04
Tuteja, N., Gill, S. S. (2013). Crop improvement under adverse conditions. 1st edn (New York: Springer).
Upadhyaya, H., Panda, S. K., Dutta, B. K. (2008). Variation of physiological and antioxidative responses in tea cultivars subjected to elevated water stress followed by rehydration recovery. Acta Physiol. Plant 30, 457–468. doi: 10.1007/s11738-008-0143-9
Uzilday, B., Turkan, I., Sekmen, A. H., Ozgur, R., Karakaya, H. C. (2012). Comparison of ROS formation and antioxidant enzymes in cleome gynandra (C4) and cleome spinosa (C3) under drought stress. Plant Sci. 182, 59–70. doi: 10.1016/j.plantsci.2011.03.015
Vanderauwera, S., Suzuki, N., Miller, G., van de Cotte, B., Morsa, S., Ravanat, J. L., et al. (2011). Extranuclear protection of chromosomal DNA from oxidative stress. Proc. Natl. Acad. Sci. U.S.A. 108, 1711–1716. doi: 10.1073/pnas.1018359108
Vanderauwera, S., Vandenbroucke, K., Inzé, A., van de Cotte, B., Mühlenbock, P., de Rycke, R., et al. (2012). AtWRKY15 perturbation abolishes the mitochondrial stress response that steers osmotic stress tolerance in arabidopsis. Proc. Natl. Acad. Sci. U.S.A. 109, 20113–20118. doi: 10.1073/pnas.1217516109
Vital, S. A., Fowler, R. W., Virgen, A., Gossett, D. R., Banks, S. W., Rodriguez, J. (2008). Opposing roles for superoxide and nitric oxide in the NaCl stress-induced upregulation of antioxidant enzyme activity in cotton callus tissue. Environ. Exp. Bot. 62, 60–68. doi: 10.1016/j.envexpbot.2007.07.006
Voss, I., Suni, B., Scheibe, R., Raghavendra, S. (2013). Emerging concept for the role of photorespiration as an important part of abiotic stress response. Plant Biol. 15, 713–722. doi: 10.1111/j.1438-8677.2012.00710.x
Wang, G. P., Hui, Z., Li, F., Zhao, M. R., Zhang, J., Wang, W. (2010). Improvement of heat and drought photosynthetic tolerance in wheat by overaccumulation of glycinebetaine. Plant Biotechnol. Rep. 4, 213–222. doi: 10.1007/s11816-010-0139-y
Wang, X., Li, Q. T., Chen, H. W., Zhang, W. K., Ma, B., Wu, W. R. (2021). Epigenetic regulation and gene expression changes associated with maize response to abiotic stress. Plant Cell Environ. 44 (1), 201–212.
Wang, X., Shan, X., Wu, Y., Su, S., Li, S., Liu, H., et al. (2016). iTRAQ-based quantitative proteomic analysis reveals new metabolic pathways responding to chilling stress in maize seedlings. J. Proteomics 146, 14–24. doi: 10.1016/j.jprot.2016.06.007
Wang, F. Z., Wang, Q. B., Know, S. Y., Kwak, S. S., Su, W. (2005). A. enhanced drought tolerance of transgenic rice plants expressing a pea manganese superoxide dismutase. J. Plant Physiol. 162, 465–472. doi: 10.1016/j.jplph.2004.09.009
Wang, Y., Wisniewski, M., Meilan, R., Cui, M., Webb, R., Fuchigami, L. (2005). Overexpression of cytosolic ascorbate peroxidase in tomato confers tolerance to chilling and salt stress. J. Am. Soc Hortic. Sci. 130, 167–173. doi: 10.21273/JASHS.130.2.167
Wang, T. Z., Xia, X. Z., Zhao, M. G., Tian, Q. Y., Zhang, W. H. (2013). Expression of a medicago falcata small GTPase gene, MfARL1 enhanced tolerance to salt stress in arabidopsis thaliana. Plant Physiol. Biochem. 63, 227–235. doi: 10.1016/j.plaphy.2012.12.004
Wang, Y., Ying, Y., Chen, J., Wang, X. (2004). Transgenic arabidopsis overexpressing Mn-SOD enhanced salt-tolerance. Plant Sci. 167 (4), 671–677. doi: 10.1016/j.plantsci.2004.03.03
Xu, S. C., Li, Y. P., Hu, J., Guan, Y. J., Ma, W. G., Zheng, Y. Y., et al. (2010). Responses of antioxidant enzymes to chilling stress in tobacco seedlings. Agric. Sci. China 9, 1594–1601. doi: 10.1016/S1671-2927(09)60256-X
Yamane, K., Mitsuya, S., Taniguchi, M., Miyake, H. (2010). Transcription profiles of genes encoding catalase and ascorbate peroxidase in the rice leaf tissues under salinity. Plant Prod. Sci. 13, 164–168. doi: 10.1626/pps.13.164
Yang, Y., Guo, Y. (2018). Unraveling salt stress signaling in plants. J. Integr. Plant Biol. 60 (9), 796–804. doi: 10.1111/jipb.12689
Yang, Y., Han, C., Liu, Q., Lin, B., Wang, J. (2008). Effect of drought and low light on growth and enzymatic antioxidant system of picea asperata seedlings. Acta Physiol. Plant 30, 433–440. doi: 10.1007/s11738-008-0140-z
Yousuf, P. Y., Hakeem, K. U. R., Chandna, R., Ahmad, P. (2012). “Role of glutathione reductase in plant abiotic stress,” in Abiotic stress responses in plants, vol. pp. Eds. Ahmad, P., Prasad, M. N. V. (Springer), 149e158.
Zarei, S., Ehsanpour, A. A., Abbaspour, J. (2012). The role of over-expression of P5CS gene on proline, catalase, ascorbate peroxidase activity and lipid peroxidation of transgenic tobacco (Nicotiana tabacum l.) plant under in vitro drought stress. J. Cell Mol. Res. 4, 43–49. doi: 10.22067/jcmr.v4i1.18249
Zhang, J., Chen, S., Li, Y., Di, B., Zhang, J., Liu, Y. (2008). Effect of high temperature and excessive light on glutathione content in apple peel. Front. Agric. China 2, 97–102. doi: 10.1007/s11703-008-0002-x
Zhang, B., Liu, K., Zheng, Y., Wang, Y., Wang, J., Liao, H. (2013). Disruption of AtWNK8 enhances tolerance of arabidopsis to salt and osmotic stresses via modulating proline content and activities of catalase and peroxidase. Int. J. Mol. Sci. 14, 7032–7047. doi: 10.3390/ijms14047032
Zhang, Y., Yang, J., Lu, S., Cai, J., Guo, Z. (2008). Overexpressing SgNCED1 in tobacco increases aba level, antioxidant enzyme activities, and stress tolerance. J. Plant Growth Regul. 27, 151–158. doi: 10.1007/s00344-008-9041-z
Zhang, Z., Zhang, Q., Wu, J., Zheng, X., Zheng, S., Sun, X. (2013). Gene knockout study reveals that cytosolic ascorbate peroxidase 2 (osapx2) plays a critical role in growth and reproduction in rice under drought, salt and cold stresses. PloS One 8, e57472. doi: 10.1371/journal.pone.0057472
Zhang, L., Zhao, G., Jia, J., Liu, X., Kong, X. (2017). Molecular characterization of the catalase gene family and its response to abiotic stress in grape (Vitis vinifera l.). PloS One 12 (11), e0188346.
Zhu, J. K. (2016). Abiotic stress signaling and responses in plants. Cell 167 (2), 313–324. doi: 10.1016/j.cell.2016.08.029
Zitka, O., Krystofova, O., Hynek, D., Sobrova, P., Kaiser, J., Sochor, J., et al. (2013). “Metal transporters in plants,” in Metal transporters in plants. Eds. Gupta, D. K., Corpas, F. J., Palma, J. M. (New York: Springer), 19–41.
Keywords: abiotic stress, reactive oxygen species, enzymatic antioxidants, non-enzymatic antioxidants, stress signaling
Citation: Mishra N, Jiang C, Chen L, Paul A, Chatterjee A and Shen G (2023) Achieving abiotic stress tolerance in plants through antioxidative defense mechanisms. Front. Plant Sci. 14:1110622. doi: 10.3389/fpls.2023.1110622
Received: 29 November 2022; Accepted: 15 May 2023;
Published: 02 June 2023.
Edited by:
Meng Jiang, Zhejiang University, ChinaReviewed by:
Muhammad Iqbal, Jamia Hamdard University, IndiaMd. Kamrul Hasan, Sylhet Agricultural University, Bangladesh
Rongbin Hu, University of California, Riverside, United States
Copyright © 2023 Mishra, Jiang, Chen, Paul, Chatterjee and Shen. This is an open-access article distributed under the terms of the Creative Commons Attribution License (CC BY). The use, distribution or reproduction in other forums is permitted, provided the original author(s) and the copyright owner(s) are credited and that the original publication in this journal is cited, in accordance with accepted academic practice. No use, distribution or reproduction is permitted which does not comply with these terms.
*Correspondence: Guoxin Shen, guoxin.shen@ttu.edu; Neelam Mishra, neelamiitkgp@gmail.com, neelammishra@sjc.ac.in