Abstract
The emergence of textile-based wearable sensors as light-weight portable devices to monitor desired parameters, has recently gained much interest and has led to the development of flexible electronics on non-rigid substrates. The flexible biosensors may result in improved sports performance, to monitor the desired bodies for injuries, improved clinical diagnostics and monitor biological molecules and ions in biological fluids such as saliva, sweat. In addition, they could help users with different types of disorders such as blindness. In this context, new composite and nanomaterials have been found to be promising candidates to obtain improved performance of the textile based wearable devices and to optimize the structures for intimate contact with the skin for better functionality. This review aims to provide the most recent cutting-edge information on emergence, fabrication, materials, and applications of chemical and physical flexible and stretchable textile-based (bio)sensors. Besides this, we discusss the recent key innovations and applications of textile-based sensors in healthcare.
Export citation and abstract BibTeX RIS

This is an open access article distributed under the terms of the Creative Commons Attribution 4.0 License (CC BY, http://creativecommons.org/licenses/by/4.0/), which permits unrestricted reuse of the work in any medium, provided the original work is properly cited.
The design and development of flexible and wearable sensor systems based on textile for measuring and quantifying physical and chemical signals generated by the human body provide an opportunity for disease diagnosis, therapy, security, robotics, and health monitoring.1–6 Current advances in textile technologies, new materials, nanotechnology, and miniaturized electronics are making wearable systems more feasible.7,8 Figure 1 shows different wearable sensors based on a textile that have attracted considerable commercial interest and vigorously expanded their scope for applications. For example, integrating sensing capabilities into textiles can provide significant benefits in diverse fields, such as healthcare, sports performance, and military issues.3,9,10 These sensors hold considerable promise for maintaining and improving the quality of life while reducing medical costs. Also, these sensors can be used to remotely control personal health status as well as the physical state of persons (e.g an athlete, a soldier etc).
Figure 1. Two main applications of textile based sensors: Chemical sensors: (A. Biomolecule analysis,11 B. Humidity sensor,12 C. Sweat analysis,13 and physical sensors: (D, Respiration rate sensor.14 E, Temperature sensor,15 F. Pressure sensor16 G. Obstacle sensor.17
Download figure:
Standard image High-resolution imageRecently, there has been an increased demand for wearable devices in the market, as demonstrated by the growth of the wearable fitness market to $34 billion in 2020 and this rate is expected to increase in future.18,19 There are a variety of sensory systems that can be used in this area. It may be noted that most of these existing methods are complex and require sophisticated equipment that may not be readily available and applicable. Thus, the development of a new, small, safe, easy to use, sensitive and rapid method of detection still remains a challenge. Among all the sensors, some electrochemical devices have been developed that enable real-time monitoring both of chemical species in biological fluids (sweat, urine, tears), explosive residues and toxic gas,20–23 and physical changes, vital human signs such as body temperature, for monitoring body position, temperature, stretching and movements.3,10,24,25 Some techniques currently exist for the fabrication of electrochemical (bio) sensors and bio-devices based on textiles and several similar flexible platforms such as a shirt, bandages, glove and belt have been proposed (Fig. 1).
The advent of a new era of the flexible sensor will be expedited by innovations in flexible electronic and power sources.26–29 Based on these findings, the new research direction of electronic flexible on textile has recently been launched. Also, more importantly, overcoming the design limitation, along with the fabrication of reliable electrochemical materials with optimized attributes and functionality, will facilitate the development of flexible and wearable devices. These smart devices can be miniaturized and equipped with remote and antenna systems for online monitoring and remote detection.30,31
Textiles represent an attractive class of substrates for fabricating wearable chemical smart sensors. The idea of wearable electronics or smart fabric will require the fabrication of devices directly into the fabric and these integrated electronic devices need to be flexible, light-weight and durable.20,32 In addition, the challenge of the wearable sensor lies in linking the computer hardware to clothing or textile sensor. Steve Mann in the 1980 s, at Massachusetts Institute of Technology (MIT) implemented this technique by attaching various kinds of electronic devices to garments.33 They used different methods to attach electrical components directly onto the fabric as shown in Fig. 2a a user has to carry heavy rigid boxes and instruments and some wires in inflexible areas with visible wires through the cloths are impractical for daily use and cause users unsatisfied and uncomfortable. Currently, most of the research on wearable electronics is focused on the attachment or fabrication of electronic devices onto the textile for example with conductive yarns and using remote or Wi-Fi systems; microcomputers make them more exciting and convenient for end-users.33,34 The intrinsic rough surface of textiles is problematic for electronic device fabrication. However, this property is ideal for many devices in which large surface roughness is actually preferred.
Figure 2. Recently developed wearable electrochemical sensors based on textile: (a) Wearable computing devices developed by Steve Mann (Reprinted with permission from Taylor & Francis, Copyrights 2016).35 (b)–(f) Fabircaiton technique, schematic of prototype electrode and illustration of a K+ sensing band-aid with the membrane-coated sensing section, respectively. The sensor shows (f) the response obtained when sensor is exposed to different concentration of K+. (Reprinted with permission from the Royal Society of Chemistry, Copyright 2013)36 (g) pH-sensitive bandage: Fabrication process and (h) potentiometric time-trace of a pH bandage sensor from pH 8.51 to 2.69 (Inset: EMF (mV) vs pH). (Reprinted with permission from WILEY-VCH, Copyright 2014).37
Download figure:
Standard image High-resolution imageConductive Materials and Fabrication Methods
One important consideration for wearable electronics is the choice of materials used in the fabrication and the possibility to provide good electrical performance with desirable mechanical properties, and stability into the flexible devices. The development of conductive and (semi)conductive materials, which are flexible, are necessary for wearable electronics. These smart materials are incorporated into the textile structure by different technologies such as weaving, embroidering, knitting, printing, chemical treatments32 and coating (coating the fibers with metals, galvanic substances or metallic salts by electroless plating and with a conductive polymers).38,39 The different materials like conductive polymers (e.g polypyrrole, polyaniline),38,40 metals and metal oxide nanoparticles/nanowires/nanorod (such as silver and zinc oxide),39 carbon-based micro/nanomaterials (such as graphene, carbon particles, and carbon nanotubes),40 have been used and investigated as textile modifier. Generally, in addition to metallic yarn and strain, smart materials can be grouped into three categories: conductive organic polymers, carbon-based micro/nanomaterials, and conductive metallic nanostructures. The following section discusses some of the materials and the fabrication technology frequently used to accomplish these goals.
Conducting polymers
Conductive polymers are organic polymers exhibiting electrical properties similar to those of metals. Polypyrrole, polyaniline, and polythiophene fibers are good examples in practical flexible and stretchable electronic devices, and they can be produced with different sizes and even in nanometer dimensions, with high conductivity (120–130 S cm−1) at room temperature.10,41,42 To provide the necessary biocompatibility and increased resistance to mechanical stress these polymers can be modified and produced in different shapes such as fiber or nanofiber. Nanofiber is a class of nanomaterials having a diameter in the submicron range (50–500 nm), small pore size, sometimes with high porosity and high surface area with length is in the micron or millimeter range such as polyaniline nanofibers.43 Due to their high surface area, they possess unique properties that make them potential candidates for a wide range of applications such as filtration, barrier fabrics, protective clothing, wipes and biomedical applications such as scaffolds for tissue engineering.44 Different methods such spin coating, printing or chemical or electrochemical treatment can be used to synthesize polymer nanofibers.45 Among these, the printing provides a convenient route to the deposition of conducting materials such as polymers with spatial resolution in the X,Y plane and makes a layer with thicknesses in nanometer dimensions. In addition, the screen-printing method provides an easily adopted fabrication route for the fabrication of wearable electronics, where all the layers with different functions can be printed on top of the fabric substrate with a layer-by-layer process. Another significant advantage of this method is that they facilitate the use of low-cost patterning techniques at room conditions.46–48 Generally, the most promising materials are organic polymers because they are intrinsically flexible or can be easily used for making desired composite materials with special chemical, physical, and electronic properties.
Carbon-based micro/nano materials
Zero, one and two dimensional carbon-based micro/nanomaterials such as graphene (nano nanosheets), carbon nanotube, carbon nanoparticles and its derivatives are promising materials for flexible and wearable electronics, due to their unique properties such as their high electron transfer rate and mechanical properties, large surface area, environmental stability and the potential for production at low cost.49
Among the various carbon materials, carbon nanotubes and graphene are two of the most intensively explored carbon allotropes in electrochemical sensors that have been evaluated as the electrode materials with great potential in wearable electronics.50 A stretchable, porous, and conductive textile has recently been developed by a simple 'dipping and drying'' process using a carbon nanotubes ink.51 Interestingly, some efforts have been made to assemble carbon nanotubes into macroscopic fibers in which the carbon nanotubes are highly aligned to maintain high mechanical strength and electrical conductivity of individual carbon nanotube. Particularly, carbon nanotube fibers are anticipated to have a broad range of potential applications, such as fiber-based microelectrodes and (bio) sensors.
Conductive metallic nanostructures
Much attention has been paid towards the preparation and applications of nanostructured coatings onto textile substrate due to their promising applications.52,53 Low dimensional nanostructures of metals, e.g., nanowires, nanorods (ZnO, Ag) or nanoparticles (such as Au, Ag, or Pt), are particularly attractive for fiber-based flexible and wearable electronics because some of these like gold and silver have very high conductivities.10,39,53–56 The nanostructured films deposited on textiles have exhibited excellent properties such as conductivity and antimicrobial activity. However, stability, is currently a big challenge and efforts are being made on improving the stability of metallic nanowires and nanoparticles on a textile substrate and utilizing them for inflexibly electronic applications.52 Moreover, nanocomposite materials are good choice, as the polymers can be combined with nanostructures that may acquire sensing capability via conductive materials printed onto fibers or fabrics. The various approaches to make fiber-based flexible and wearable electronics by using conductive metal(s) can be grouped into two categories. In the first one, the electronic textile can be fabricated by using conducting fibers made from conductive metal such as steel. While the second category is thin-film-based electronic components where the different methods such as electro-spinning, wet spinning, spin coating, printing or chemical treatment (hydrothermal method) are being used for the preparation of materials. The printing provides a convenient route to the deposition of conducting nanocomposite materials with different thicknesses and the desired materials can be printed on top of the textile substrate through a layer-by-layer process.10,46,53,57 In all cases, the optimal textile candidate for supporting printable electrochemical sensors have been predicted to have inert properties and yield stable operation for extended periods under normal and heavy wear.
Applications of Textile Sensors
Along with flexible electronic, textile electronics and its applications in flexible sensors are attractive for many applications. Textiles represent a striking class of substrates for fabrication of wearable biosensors. Smart textiles describe the convergence of electronics and textiles into fabrics that can be used to sense, compute, communicate and actuate. Wearable biosensors ideally should have the ability to monitor the physiology of the wearer and the surrounding environment. The integration of chemical sensing into textiles thus permits the wearer to receive extremely valuable and timely information without compromising the functionality or comfort of the garment.3,10,20,58–60
The sensors can be divided into active and passive sensors. The passive sensors require an external power source, while active sensors can convert the input energy (elastic, thermal, etc) into a measurable difference of potential. The majority of textile sensors are known to be passive. Also, all of these systems require a power supply to function.34,61,62 Because of the portable nature of these devices; batteries can be employed in almost most of them. At first batteries were unfixable. They could not be fully integrated with smart textiles. Developing flexible batteries is seen as an indispensable enabling technology for smart textiles and wearable sensors. The computers can be coupled to smartphone platforms directly and all wires can be eliminated.63–65 We will discuss the recent advances and developments in the field of textile or wearable sensors with emphasis on two subclasses of these sensors i.e. chemical and physical wearable sensors.
Chemical wearable sensors based on textile
The chemical wearable sensors include (bio) electrochemical and optical and gas sensors where a chemical reaction with the participation of the analyte (ions or (bio) molecules) gives rise to the analytical signal (Figs. 2 and 3). The gas sensors classified into chemical sensors are based on change in the conductometry. Wearable (bio) chemical systems can be used for health monitoring, military applications etc.62 Among the different sensors, electrochemical (bio) sensors have a unique position for addressing the needs for field screening and identification of various chemical from ions to gas molecules. They have been found to be suitable for manufacture and miniaturization. Simplicity, low-voltage operation and low-power consumption of electrochemical sensors make them suitable for the requirements of on-body wearable systems into clothes. They are compatible with diverse materials and can operate with inexpensive battery-powered electronic instrumentation.61,62 Electrochemical wearable sensors based on textile are being used for the detection of ions (Na+, H+, Ca2+, NH4+, etc) (Figs. 2 and 3), biomolecules (glucose, lactate and uric acid) in different body fluids (sweat and saliva) and volatile compounds (such as: CH4, NO2, NH3, CH3OH, (CH3)2CO and body odor) typically present in a body or in our environment (Table I).
Figure 3. Recently developed wearable electrochemical sensors based on textile: (a) Wearable screen-printed electrochemical sensors on underwater garments and scanning electrode image (b) representing the working electrode area. (c) Chronoamperograms of the tyrosinase-modified neoprene SPE in the presence of different concentrations of catechol samples and corresponding calibration curves (inset). (d) Stability of the signal over time with respect to the initial measurement: (i) 10.0 mM 4-chlorophenol and (ii) 2.0 mM phenol (Reprinted with permission from Taylor & Francis, Copyrights 2016)35 (e) Screen-printed carbon electrodes on the underwear. Single electrode morphology (right inset) linear-scan voltammetric response for increasing NADH concentrations over the 0.0–100.0 mM range (left inset) are also presented. (Reprinted with permission from the Royal Society of Chemistry, Copyright 2013).36 (f) Screen-printed textile-based stretchable multi-ion (K+ and Na+) potentiometric sensor with its response(g) to different concetrations of K + (red) and Na + (blue) ions (EMF (mV)/time (sec). (Reprinted with permission from WILEY-VCH, Copyright 2014).37
Download figure:
Standard image High-resolution imageTable I. (Bio) electrochemical and optical and gas wearable sensors based on textile.
Sensor | Analyte | Clothing/the role of textile | Sample(s) | Sampling Method of sensor | Measurement technique | Sensing parameter | References |
---|---|---|---|---|---|---|---|
Optical | pH | clothing, head- or wristbands | Sweat | Textile channel | Colorimetric/LED/indicator | pH range: | 66 |
2.0–8.0 | |||||||
Optical | H2O2, pH | — | — | Textile channel | Colorimetric | pH: 3.0–7.0 | 67 |
LDR: 0.1–0.6 μM | |||||||
Optical | pH | waistband | Sweat | Textile channel | Colorimetric/indicator array | pH range: | 68 |
4.5–8.0 | |||||||
Optical | pH | Silk fabric – Textile sensor | Polluted water | — | UV–vis reflectance absorption spectra | pH range: | 69 |
2.0–10.0 | |||||||
Optical | Glucose/ Temperature | Cotton fabric- Shirt | Sweat | Textile channel | Colorimetric | LDR:50–600 μM | 70 |
LOD:13.45 μM | |||||||
Optical | Levofloxacin | — | — | On Body | Surface-enhanced Raman spectroscopy | N.R. | 71 |
Optical | pH and lactate | Cotton fabric | Sweat | On Body | Colorimetric | pH: 1.0–14.0 | 72 |
LDR:0.0–25.0 mM | |||||||
Optical | Ammonia and Hydrogen chloride | Cotton Fabric treated with chemical indicator | Air | — | Image analysis | LDR: 50–1000 ppm | 73 |
Electrochemical | Lactate | woven electrode into textile | Sweat | On Body | Amperometric | LDR: 4.0–20.0 μM | 74 |
Electrochemical | Blood leakage sensor | Cotton | Blood | On Body | Impedance | LVOD: 15 μl | 12 |
fabric. | |||||||
Electrochemical | Nitro-aromatic Explosives | screen-printed electrodes on textile | Air | — | Voltammetry | LDR: 0.0–50 ppm | 20 |
LOD: 1.0 ppm | |||||||
Electrochemical | lactate | Cotton | Salvia | — | Amperometric | LDR: 0.1–5.0 mM | 21 |
fabric. | LOD: 0.3 mM | ||||||
Nitrile glove | |||||||
Electrochemical | Phenolic contaminants, TNT, Cu2+ | underwater garments | Marine environments | On Body | Amperometric, voltammetry | [TNT]: | 75 |
LDR:100 − 900 ppb | |||||||
LOD: 42 ppb | |||||||
[Cu2+]: | |||||||
LDR:10–90 ppb | |||||||
LOD: 13 ppb | |||||||
[4-chlorophenol]: | |||||||
0.0–25.0 μM | |||||||
LOD: 0.43 mM | |||||||
[Phenol]: | |||||||
LDR: 1.0–5.5 μM | |||||||
LOD: 0.25 mM | |||||||
Electrochemical | NADH and H2O2 | screen-printed electrode on the underwear | Sweat, and Salvia | On Body | Amperometric | NADH: | 76 |
LDR: 0–100 mM | |||||||
LOD: N.R. | |||||||
H2O2 | |||||||
LDR: 0–25 mM | |||||||
LOD: N.R. | |||||||
Electrochemical | Na+, K+, Cl–,Cd2+, Pb2+, pH | textile-based sampling | — | Textile channel | Potentiometric | [Ions]: | 77 |
LDR: 10−7−10−1 M | |||||||
Electrochemical | pH, K+, NH4+ | band-aid/conductive yarns coated with polymeric | Sweat | On Body | Potentiometric | pH: 3.0–11.0 | 36 |
membrane | K+ | ||||||
LDR: 0.01–100 mM | |||||||
LOD: 10.0 μM | |||||||
NH4+ | |||||||
LDR: 0.001–10 mM | |||||||
LOD: 1.0 μM | |||||||
Electrochemical | pH | Bandages/screen-printed | Sweat | On Body | Potentiometric | pH: 3.0–10.0 | 78 |
pH potentiometric sensor | |||||||
Electrochemical | Na+,K+ | screen-printed | Sweat | On Body | Potentiometric | [Na+] and [K+]: | 13 |
potentiometric sensor | LDR: 0.1–100 mM | ||||||
LOD: 10–4.9 | |||||||
Electrochemical | Glucose or lactate - | Polyester(T- Shirt) | Sweat | On Body | Amperometric | Glucose | 11 |
LDR: 0.0–40 mM | |||||||
LOD: N.R. | |||||||
Lactate | |||||||
LDR: 0.0–50 mM | |||||||
LOD: N.R. | |||||||
Electrochemical | Uric acid | Bandage/ | Sweat | On Body | Amperometric | LDR: 100.0–800 μM | 22 |
screen-printed | LOD: N.R | ||||||
potentiometric sensor/ | |||||||
wireless | |||||||
Electrochemical | Adrenaline | Cotton yarn functionalised | Sweat | On Body | Oxidation of adrenaline at the Pt-gate electrode | LDR: 0.001–1.0 mM | 79 |
with Organic electrochemical transistors | LOD: N.R | ||||||
Electrochemical | Cl− | polyester | Sweat | On Body | Potentiometric | LDR: 10.0–100 mM | 80 |
LOD: N.R | |||||||
Electrochemical | Na+ | carbon fibres integrated into wearable patch, garments | Sweat | On Body | Potentiometric | LDR: 1.0–100.0 mM | 81 |
LOD: N.R | |||||||
Electrochemical | Interleukin-6 | Textile coated with layers of silver and carbon | blood | — | Stochastic microsensors | LOD:1.0 fg. mL−1 | 82 |
Electrochemical | DMP, NH3, | polymers | Air | — | Conductometry | N.R. | 83 |
NO2 | coated Nylon | ||||||
Electrochemical | NH3,HCI | PPy-coated textile fabrics | Air | — | Conductometry | N.R. | 84 |
Electrochemical | Solvent vapors | Carbon black/polymr gas sensors integrated into a textile | Air | — | Conductometry | Acetone | 85 |
LDR: 50.0–500 ppm | |||||||
LOD: N.R | |||||||
Electrochemical | NH3 | Modified commercial yarns with polyaniline | Air | — | Conductometry | — | 11 |
Electrochemical | influenza A virus | A textile screen-printed biosensor on polyamide substrates | Biofluid | On body | Potentiometric | LDR: 10 ng ml−1 to 10 μg/ml | 86 |
LOD: 10 ng ml−1 | |||||||
Electrochemical | Volatile amine | Nano-polymeric composite | Body odor | On body | Conductometry | LDR:50–1000 ppm | 23 |
Abbreviations: [TNT]: 2,4,6-trinitrotoluene,[ DMP]: Dimethyl methylphosphonate, [PPy] Polypyrrole, [LDR]: Linear dynamic range, [LOD]: limit of detection, [NADH]: Nicotinamide adenine dinucleotide, [N.R].: Not reported.
In this kind of sensors, voltammetric, potentiometric, and amperometric techniques can be used. These sensors and their parts can be embedded into or on textiles such as in belt, arm hand, shirt, underwater garment, bandages and jacket and etc.61,75,76 Numerous sensors based on textiles, cloths or yarns that show potential for integration with clothing can be used as (bio) sensor. Table I shows the characteristics and analytical performances of the textile electrochemical (bio) sensors. The researchers have tried to design the architecture of the removable sensor to eliminate problems commonly associated with the washing of integrated textile sensors. For fabricating clothes with electrochemical sensors, the direct screen-printing of electrodes on the textile substrate is convenient and straightforward technique.20,46 However, it depends on differences in the hydrophobicity of various textile substrates such as cotton, polyester, and Gore-Tex (waterproof, breathable fabric).20 Owing to the extreme hydrophobicity exhibited by the Gore-Tex fabric in comparison with other natural, synthetic fabrics, electrochemical sensing is facilitated; it has been shown to be a promising substrate for thick-film electrochemical sensors. Another attractive strategy was reported by J. Wang et al.36 wherein the cotton yarns (Fig. 2b) were immobilized with carbon nanotube ink and it was used as a potentiometric sensor for detection of pH, K+, and NH4+. Finally, they could fabricate a novel pH-sensitive bandage (Fig. 2g). The potentiometric time-trace of a pH bandage sensor from pH 8.51 to 2.69 along with EMF dependence vs pH for the PANI conducting polymer ISE is shown in Fig. 2h.
In sports (Figs. 3a–3f), bioelectrochemical21,76,74 textile sensor plays a critical role. Biosensors are closely related to chemical sensors, distinguished by the molecular recognition element which is biological in nature (e.g. enzyme). The glucose68 and lactate74 sensors based on textiles have also been investigated. Figures 3a–3d are typical chronoamperograms of the tyrosinase-modified neoprene SPE exposed to catechols (such as 4-chlorophenol and phenol) and the stability of the signal over time with respect to the initial measurement for proposed sensors, respectively. As can be seen they developed flexible electrochemical sensors on underwater garments that are able to detect trace levels of marine pollutants and explosive threats in seawater. The electrode response stability was examined over 50 min, relative to the initial measurement for (i) 4-chlorophenol and (ii)phenol. These tests yielded a low RSD for each compound, ranging from 2.07% to 1.73%, respectively.
By using smart textile sensors, it is estimated that 70% of all illnesses are preventable, if suitable screening measurements could be introduced, this could produce dramatic reductions in costs for treatments and medication.3,87 For example, by adopting suitable exercise and diets, many lifestyle-related illnesses can be prevented. Exercise generates sweat which contains rich information about the physiological condition of the subject as it contains a matrix of essential ions and molecules.36,78 Real-time sweat analysis (Figs. 3f–3g) during exercise can give valuable information on dehydration and changes in the number of essential biomolecules and ions such as K+ and Na+ ions. Sweat, saliva, and tears contain multiple physiologically relevant chemical constituents and can be easily obtained continuously for non-invasive real-time monitoring of these analytes. For example, by monitoring level of electrolytes (pH, sodium, ammonium, calcium) and metabolites (glucose, lactate, urea) in sweat and the skin interstitial fluid with suitable sensors can provide valuable health information.88,89
Despite the extensive progress made in wearable electrochemical (bio)sensors for physiological monitoring, military application, and sports, little attention has been directed at the optical wearable (bio)sensors,68,67 due to the difficulty for use in optical systems and related instruments into textiles and clothes. These sensors require the desired sample for analysis. Usually, a body fluid such as sweat is delivered to the sensor active surface, after which a reaction occurs, and a signal is generated in a few minutes in these sensors, textiles can act as a micro pump. For example, Schazmann et al.88 reported a smart belt that electrode set on textile and it was located on the body to monitor Na+ ions in sweat in real time. In this sensor, textile plays the role of sweat pump to the sensor area.
In the optical sensors, a colorimetric chip based on iono-gel (Fig. 4a) has been developed for monitoring pH of sweat.68 There are many factors that can be correlated with sweat pH and health. Changes in the pH of the skin are reported to play a role in the pathogenesis of skin diseases like irritant contact dermatitis and acne, and also, showed that inducing metabolic alkalosis through the ingestion of sodium bicarbonate may lead to increased blood and sweat pH. In addition, it has been reported that sweat pH rises in response to an increased sweat rate. Schematic illustration of the micro-fluidic system's performance over and structure of the probe is shown in Figs. 4a–4c. Accordingly, The probe is applied to the monitoring of the pH of sweat during an exercise time. It was the first wearable sweat sensor with an ionogel being an important component in the fabrication of the sensing platform. The ionogel plymer provides an ideal substrate for the organic pH indicator dyes (bromophenol blue, bromocresol green, bromocresol purple and bromothymol blue). It could be attached onto the textile and could be used for monitoring the pH from 0 to 14 in intervals of one pH unit (Fig. 4c). This sensor showed no leaching of the indicators during experiments, the prolonged stability of the device for the monitoring of sweat pH measurements over time and the micro-fluidic structure (Fig. 4a) guarantees that freah sweat is continuously transferrd from the skin to the ionogels.
Figure 4. Recently developed wearable optical and optoelectronic sensors based on textile: (a), (b) Schematic illustration of the micro-fluidic system's performance of wearable sweat sensor over time c) Microfluidic system integrated into a wrist-band (Reprinted with permission from Elsevier, Copyright 2012).68 (d) pH sensing patch (top) and fabric passive pump design (down) (e) pH and (f) sweat rates measured during a 60 min effort of two volunteer (Reprinted with permission from IEEE, Copyright 2009).66 (g) shematic representation of a colorimetric sensor with (h) a standard color charts and real-time sweat pH and Lactate monitoring based on a textile platform. (i). Standard color charts of the textile based pH sensor (a) and L* a* b* color model of pH in 0.1 M PBS solution (b). (Reprinted with permission from Elsevier, Copyright 2019).72
Download figure:
Standard image High-resolution imageShirley Coyle et al.66 reported a new optoelectronic probe on textile using a simple photometer (Fig. 4d) where a textile-based platform with fluid handling properties was used to collect and analyze the sweat samples. A fabricated channel is created by screen printing an hydrophobic acrylic paste on either side of the fabric. At one end of this channel is a window where sweat enters from the skin, based on capillary flow through the fabric. For sensing pH, a sensitive dye (bromocresol purple) is immobilized onto the channel (Fig. 4e). The dye for sweat analysis exhibits color changes from yellow to blue over this pH range A paired emitter-detector LED was used to quantify the color change of the dye. The fabricated optoelectronic textile based sensor was embedded into a waistband as a model and tested on the body during exercise succefully.
Recently, N. Promphet and et. al.72 developed a textile-based colorimetric sensor for the detection of lactate and sweat pH and by depositing three different layers (chitosan, sodium carboxymethyl cellulose and indicator dye for pH sensing for lactate assay) onto a cotton fabric (Fig. 4g). The sensing probe for pH was composed of a mixture of two organic dyes (methyl orange and bromocresol green). As the pH of sweat from 1−14, the pH indicator shifted from red to blue, whereas the purple color intensity was enhanced by increasing lactate concentration (reange 0–25 mM) in the sweat (Figs. 4h–4i). The proposed sensor was tested on three human volunteers before and during jogging successfully.
Physical sensors based on textile
The physical wearable sensors (temperature, pressure, obstacle and strain sensors) can be fabricated using textiles (Fig. 5). In general, a physical sensor, defined as a device that detects a change in a physical stimulus, turns that stimulus into a signal that can be measured or recorded.34,78
Figure 5. Recently developed wearable physical sensors based on textile: (a) An schematic of the encapsulation for a thermistor within a yarn and photograph of prototype sock (b) fabricated with electronic temperature-sensing yarns. (c) temperature response of three different sensors and Computer interface (d) using LabVIEW (Reprinted with permission from MDPI, Copyright 2017).90 (e) Wearable obstacle detection system fully integrated into textile structures worn by the mannequin moving towards an object (f) recorded signals over the 1st, 2nd and 3rd vibration motors on the left and right arms during the movement towards front obstacle (Reprinted with permission from Elsevier, Copyright 2012).91 (g) The schematic of the pressure sensor integrated into the bandage as a substrate for detection of articular and muscular movement: finger (h) and knee(i) bending.24
Download figure:
Standard image High-resolution imageThese sensors have drawn considerable attention, especially in medicine and sports. With the increase in the size of the elderly population, at the same time the emergence of chronic diseases because of the changes in lifestyle, there is a need to monitor the health status of individuals in their daily routine lives. The regular activities of the human body generate various physical stimuli, such as body temperature, blood pressure, the pulse skin and strain. On the other hand, physical sensors are capable of measuring these types of physical parameters or health indicators.34 Generally, the four main vital signs routinely monitored by medical professionals are:
- i.Body temperature (exploration of infection and fever).
- ii.Heart rate (exploration of cardiovascular diseases).
- iii.The rate of breathing or respiration rate (it may increase with fever, illness, and with other medical conditions, the typical respiratory rate for a healthy adult at rest is 12–20 breaths per minute).
- iv.
Researchers have fabricated a smart T-shirt that functions to monitor the wearer's heart rate, respiration, temperature, and other vital signs.9 The Wearable Health Care System project has developed a wearable garment that can be worn under regular clothing and is capable of recording biomechanical variables and physiological signals.33,92,93 In addition, motion signals were monitored because they can be used to extract important physiological information such as the stress level or movement of a person (in some research such as research on Parkinson's disease is very useful). In all of them textile and clothes can play critical roles, and body parameters can be detected with physical wearable sensors based on textiles.
Temperature sensor
The body temperature is regarded as the first vital sign. An increase and decrease of body temperature can be due to an infection or fever and low blood flow rate (due to circulatory shock), respectively. Generally, body temperature is about 32 °C, and for measuring the temperature, the suitable places are the arm or the chest.3,7 The textile-based sensor can be used as a temperature probe based on thermistors (in thermistor electrical resistivity changes with variation of temperature). Generally, in these sensors the following formula has been used.94,95
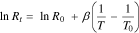
In this equation, a linear relationship between ln (Rt) and 1/T is established. Here, β (in Kelvin) is the material constant for the thermistor (it can be a metal oxide, carbon nanotubes, graphene, nickel oxide).95–98 Rt is the resistance at temperature T, and R0 is the resistance at T0. β with a temperature coefficient of the thermistor have main roles in determining the sensitivity of the thermistor.95
The thermistors should have high static and dynamic accuracy in the human skin temperature range (30 °C–45 °C) and can be embedded into or attached on textiles. Detection should be linear with possibly high-temperature efficiency and resistant to humidity is expected as an environmental challenge.99,100 Jung et al. recently found that combination of poly (3,4-ethylene dioxythiophene) poly (styrene sulfonate) (PEDOT:PSS), silver nanoparticles (AgNPs), and graphene inks can be used to fabricate a highly stretchable self-powered temperature sensor. Also, the printing technique with commercialized thermoelectric materials was utilized on stretchable knitted fabric with excellent elasticity as an affordable and scalable process. In fact, temperature gradients over the printable maters can be fabricated to control the current via the 'Seebeck effect''.101 Hence, temperature difference across the device is detected automatically and without need to any power supplier.15 In another approach, T. Hughes-Riley et al.90 reported a new electronic temperature-sensing yarn that was well-suited for making long-term monitoring of skin temperature when incorporated into a textile with a remote system (Fig. 5a). The miniatured sensor were suitable for wearable applications. They implanted a commercial thermistor chip into the fibers of a yarn, which can be used to produce textile. To protect the thermistor, it was encapsulated in a resin. The fabricated textile showed a short response time over the temperature range of interest (between 0.01–0.35 s). Finally, they could make socks (Figs. 5a–5d) as a prototype temperature-sensor and it was suitable for diabetic patients.
Obstacle detection system
The detection of obstacle in an environment of person is highly desired, in order to detect obstacles, different systems such as Radio Frequency Identification (RFI), Navigation Assistant for Visually Impaired (which was designed to convert images captured by a camera into verbal messages), GPS (Global Positioning Systems), Robotic, etc, can be used. These sensors can guide the users to detect obstacles such as a table, stairs, and even a hole.91,102,103 Bahadir et al.91 developed an innovative wearable obstacle detection system fully integrated to textile structures (Fig. 5e–5f), that enabled detection of obstacles for visually impaired people. This smart clothing included ultrasonic sensors, vibration motors (Fig. 4e), power supplies and a microcontroller. The prototype (Fig. 4e) has been tested for detection capability, power consumption, heating behavior, and wash-ability. Results have shown that developed system is able to identify the obstacle's position without any failure within its detection range around its users. Interstingly, the developed system is capable of detecting right, left and front obstacles position accurately and giving fast signal. While detecting obstacles such that when obstacles are at the left, system gives an output turn right and reverse and when obstacle is at the front, the smart cloth gives an output like turn left and right at the same time, so user can recognize there is an obstacle in front of the user. For example, Fig. 4f shows signals of vibration motors (1st, 2nd and 3rd) on the left and right arms during the movement towards an obstacle at distance about 15 cm to 1.25 m located at the left side.
Mocanu et al.17 designed and fabricated a flexible textile-based obstacle detector using paper to attain a promising wearable device for both indoor and outdoor activities. In fabricated device, two independent sections of ultrasonic sensor and internal video camera were utilized to identify both static and mobile substances, irrespective of their dimension, shape or location and interpret and notify users about coming obstacles.17
Pressure sensor
A textile-based pressure sensor has been explored for a variety of applications to include sports, caring for the elderly, diagnostics and human motion detection due to their integrability into clothes, socks, shoes, pillow, belt, headband, and even beds102,104 (Fig. 4c). In all these sensors, the determination of pressure regions or sensor positions for example in health or sports cases are very important. As we know under the human feet or shoes, different pressure regions are available.
For measuring pressure, many types of sensors such as capacitive,105 piezoresistive,106 piezoelectric,107 and optical108 have been developed. Among these, a capacitive pressure sensor has advantages in terms of its simple design, low power consumption, acceptable sensitivity, and high stability. A capacitive pressure sensor makes use of the principle of a parallel plate capacitor, which consists of two compliant conductive layers (such as conductive fabrics) and flexible spacers (flexible dielectric such as foams, fabric spacers and soft polymers). The amount of electrical charge (Q) that can be stored between the two conductive plates of such a capacitor at a given voltage V is defined by the capacitance C of the capacitor. C can be expressed as a function of the distance d, the given area A and the material between the two plates, therefore, C can be defined as:

where, ε0 (ε0 = 8.854 × 10−12 F · m−1) is the vacuum permittivity and εr is the relative dielectric constant of the dielectric material. In order to improve the performance and efficacy of the capacitive sensor Many efforts have been made for the selection of a suitable interlayer material or modification with nano-conductive materials (metallic nanoparticles, such as silver nanoparticles as filler or doping agent), and enlarge the deformation space and extend the surface area of the electrodes.109 A textile capacitor has been fabricated using two electrodes of conductive woven textile separated by compressible spacer material. Meyer,110 developed a pressure sensing pillow with several integrated textile capacitive pressure sensors made from a 3D knit fabric spacer material and conductive textile filaments coated with silver. The developed sensor could be used to detect pressure with pressures up to 10 N cm−2. An ideal pressure sensor must ensure repeatable measures over time with a low hysteresis value. Therefore, the textile pressure sensors are applicable in areas where high accuracy is not required.110
In a similar work, a smart adhesive bandage24 for monitoring human movement was developed (Figs. 4g–4i). For the fabrication of electrode of the pressure sensor, silver nanowire mixure dispersed in ethanol was drop-coated on to a polyethylene terephthalate film substrate to produce conductive networks. To monitor human movement, the PET film substrate was replaced by an adhesive bandage. Figure 4g shows the schematic of pressure sensor with low weight and high flexibility. The final sensor as a dielectric interlayer demonstrates high sensitivity, as 5.54 kPa−1 (p < 30 Pa) and 0.88 kPa−1 (p > 30 Pa), The adhesive bandage sensor was used as a substrate for detection of articular and muscular movement such as finger and knee bending (Figs. 4h–4i).
Heart rate (pulse) sensor
Traditionally, heart rate can be measured from the radial artery at the wrist and monitoring of blood pressure is performed in a clinic with trained personnel by mounting inflatable pressure cuffs with stethoscopes to the patient's arm, the so-called auscultatory method.7,10 Heart rate depends on cardithe ac cycle (the cycle is the sequence of events that occurs when the heart beats that deoxygenated blood through the lungs and pumping newly oxygenated blood). The heart rate is the frequency of cardiac cycles, expressed as beats per minute (b.p.m.). To measure the heart rate more accurately, electrical sensors can be used. Generally, skin electrodes are used to pick up the depolarization signal from the heart muscles. This technique is called as electrocardiography.111,112 A few sensors based on textile have been reported although a lot of research-based on wearaa ble sensor was reported,99,100,113
Yoo and coworkers(100) designed a wearable electrocardiogram acquisition system implemented with planar-fashionable circuit board on a shirt. The proposed wearable continuous electrocardiography monitoring system is composed of two parts: a group of electrodes and a monitoring chip. The electrodes werescreen-printed directly on fabric. Unlike conventional wet electrode, to overcome these drawbacks of conventional wet electrodes (dry out of electrolyte gel, unpleasant using of gel for a long time and also reduce of toxicity of gel) for long-term monitoring, dry electrodes can be considered as a good alternativece to the wet electrode.
Lee et al.113 developed a non-contact electrocardiogram measurement system on a bed using conductive textiles for long-term monitoring of heart activities. At first, their goal was to design and develop more user-friendly system by configuring the array and size of electrodes with no constraints of posture and position in a bed, for this purpose they used a foam layer to provide comfort and follow the curvature of the body. Their results suggested that the capacitive coupled textile system was suitable for daily healthcare monitoring and abnormal state of sleeping. Finally, they tested the system to find the potential for monitoring the sleep stages, which is essential for observing the physical and psychological states.
To obtain a continues monitoring of electrocardiogram (ECG) for possible risk of cardiac arrest in babies, Coosemans et al. (114) developed an alternative to conventional ECG electrodes to be used in the long term with no skin irritation and allergic contact reactions. Figures 6a–6c shows the integrated circuitry and secondary coil and a sample set-up with the belt prototype on a three months old baby. General system overview is depicted in Fig. 6a. The measured ECG results of new electrode called Textrode demonstrated the lower frequent drift according to baseline along with a lower signal-to-noise ratio in comparison to conventional gel electrodes. Also, the reported heart rate accuracy is a beat-to-beat detection which suffices the application.114
Figure 6. Textile based sensors: heart rate sensor (a) general sensor performance with correspond (b) ECG measurment (c) Detail of the backside of the baby suit, showing the embroidered coil and the flexprint (114). T-shirt with respiration rate sensor: (d) Prototype of the spiral antenna integrated into a cotton shirt supported by SEM image (top right) (e) Resonant frequency shift of the as a function of the induced stretch in off-body scenario.115
Download figure:
Standard image High-resolution imageRespiration rate sensor
The respiratory rate is a vital sign with an under appreciated significance that can, in acute situations, prognosticate patients' mortality rate and need for invasive ventilation. There are many approaches for respiration monitoring, common sensors can respond to the flow of breath, but in physical sensors for respiratory rate based on textile, to the expansion and contraction of the chest during breathing can be monitored. The strain or pressure sensing can be accomplished by monitoring the volume changes of the chest.3,30,34,116
The respiration rate sensors take advantage of conductive textile technology to build the sensing elements directly into the textile.116,117 Huang et al.117 knitted nonconductive fibers with conductive silver-coated to form resistive strain sensing textiles and used it as reparation rate sensor. As the textile is stretched, the contact area between the conductive fibers is reduced, and the resistance increases.
Multiple textile-based pressure sensors (capacitive sensor) have also been reported. Hoffmann et al.118 and Min et al.119 fabricated a compressible textile wherein the foam material was sandwiched between two conductive textile electrodes. In another work, they simplified structural textile capacitive respiration sensor for respiration monitoring system. In this sensor whenever the chest expands, the pressure applied on the compressible textile reduces its thickness, thus increasing the capacitance between the electrodes.
Importance of sensor comfortability for user was also emphasized by Guay et al.115 by proposing a prototype wearable designed sensor to monitor the breathing rate without any direct skin interaction or add-on electrodes (Figs. 6d–6e). Their design was multi material fibers with form of spiral antenna detecting shift of central frequency coming from the lung volume variation and strechability of chest textile, which remotely records the respiratory information in real-time.115 They reported that bearthing detection can be either due to changing the antenna configuration as result of mechanical force or changing the dielectric of human torso during respiration. The measured frequency shift for deep and shallow breathing is located in the ranges of 120–200 MHz and 10–15 MHz, respectively. As can be seen in Fig. 6d, linear line of 5–16 mm stretch range provides the respiration sensor sensitivity of 1.4 MHz mm−1, and 5% chest expansion causes 16.2 resonant frequency shift.
Conclusion
The emergence of wearable textile-based sensors has opened a brighter future to a wide range of healthcare applications. In the last decades, the considerable progress in textile materials and fabrication techniques of sensors and flexible devices have revealed the importance of these sensing platforms. The wearable textiles-based sensors can be integrated with daily clothing However, there are still exist many challenges to be addressed in terms of fabrication, design, device operation and implementation. Despite the huge progress in 'engineering'' part of wearable snesors, many key challenges such as quantification analysis and stability need to be optimized. For example, the electrochemical methods need to be improved since the obtained signal is required to be compared with either a calibration curve or a standard method. Another serious issue is the stability of the wearable sensors particularly electrochemical sensors due to passivation and biofouling of the electrode surface, which possibly increases the risk of wrong response during operation. This problem becomes more important when the enzymes or biological recognition agents are used, especially during on-body measurements. Additionally, challenges relared to power sources still remain for these types of sensors. In fact, an ideal power source should have a small size and produce stable and enough energy, flexible to increase comfort and should be close to the sensor. So more robust and stable sensing mechanisms and components must be designed and should be used to enable accurate measurements for increased chemical and mechanical stability and long-term use. In addition, although nanomaterials have been found to play an important function in flexible sensors for enhanced sensing performance, their potential towards biocompatibility and toxicity are needed to be considered and investigated.
Addressing these problems and challenges in these areas are critical for the future success of the wearable sensors. It should be noted that most sensors are used for continuous monitoring of parameters related to health and user safety.Efforts should be made to explore the applications of these sensing devices in forensic areas and drug toxicology that are still underexplored. Indeed, we predict exciting development towards the understanding of operational flexible textile-based sensors platforms for new applications. Finally, we hope that the revolution in textile sensors will lead to produce new products that can be released in the markets for our improved daily lives.
Acknowledgments
Amir Hatamie and Abdolreza Simchi are thankful for the financial support from Sharif Universityof Technology (Grant QA970816) and Iran National Science Foundation (INSF Grant 95-S-48740). Also, the authors thank Prof. Yogesh Singh, Vice Chancellor, Delhi Technological University (DTU), Delhi, India for his interest in this work. SK (DST/INSPIRE/04/2017/002750) and CMP (DST/INSPIRE/04/2015/000932) acknowledges Department of Science and Technology, New Delhi, India for DST-INSPIRE Faculty Award. B.D.M. thanks Science & Engineering Research Board (Govt. of India) for the award of a Distinguished Fellowship (SB/DF/011/2019).