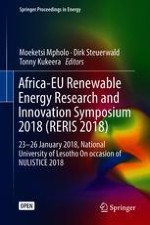
Open Access 2018 | OriginalPaper | Chapter
9. Simulation and Optimization of Renewable Energy Hybrid Power System for Semonkong, Lesotho
Author : Leboli Z. Thamae
Published in: Africa-EU Renewable Energy Research and Innovation Symposium 2018 (RERIS 2018)
Publisher: Springer International Publishing
Activate our intelligent search to find suitable subject content or patents.
Select sections of text to find matching patents with Artificial Intelligence. powered by
Select sections of text to find additional relevant content using AI-assisted search. powered by