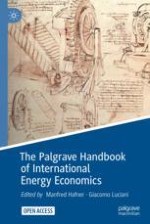
Open Access 2022 | OriginalPaper | Buchkapitel
12. Power Generation from Tides and Waves
verfasst von : Dhruv Bhatnagar, Danielle Preziuso, Rebecca O’Neil
Erschienen in: The Palgrave Handbook of International Energy Economics
Aktivieren Sie unsere intelligente Suche, um passende Fachinhalte oder Patente zu finden.
Wählen Sie Textabschnitte aus um mit Künstlicher Intelligenz passenden Patente zu finden. powered by
Markieren Sie Textabschnitte, um KI-gestützt weitere passende Inhalte zu finden. powered by